Influence of chloride ions on the stability of PtNi alloys for PEMFC cathode
Electrochimica Acta(2011)
摘要
The dependence of the rate of Ni dissolution from PtNi alloys on the chloride concentration was studied electrochemically in 0.5 M HClO 4 at room temperature. Electrodeposited PtNi catalysts were subjected to extensive potential cycling between 20 mV and 1.3 V at various Cl − concentrations and the cyclic voltammograms (CV) response and the oxygen reduction reaction (ORR) activity of the catalysts were determined at different intervals. Energy dispersive X-ray spectroscopy (EDS) and inductively coupled plasma spectroscopy (ICP) analyses were carried out to determine the elemental composition of the alloys and the amount of dissolved Ni at different stages of the potential cycling. It was found that the presence of Cl − increases the rate of Ni dissolution and by this accelerates the dealloying process relative to potential cycling in chlorine-free solutions. Dealloying is most pronounced during the initial stages of potential cycling. Already a small amount of Cl − is sufficient to dissolve the majority of the non-noble metal from the alloys. Even then, under oxygen reduction conditions, the blockage of Pt surface by Cl − is less pronounced for the alloys than for pure Pt catalysts, leading to marginally improved ORR activity for the PtNi alloys at low Cl − concentrations. From a practical point of view, the effect of chloride ion leakage from a commercially available saturated KCl reference electrode on the electrocatalytic activity was also investigated. Keywords PtNi alloys Chloride ion PEMFC contamination ORR activity Fuel cells 1 Introduction Air is the most practical and feasible oxidant for the proton exchange membrane fuel cell (PEMFC) cathode and, accordingly, impurities in air will be of major concern for the long-term performance of PEMFC systems. Airborne impurities such as SO 2 , NO 2 , H 2 S, and NO have already been identified as poisons for the Pt catalyst with irreversible damage to the cell performance even if present in minute quantities [1–4] . Similarly, anions like chloride that are introduced into the membrane and electrode assembly (MEA) through the water-cooling systems/humidifiers or residual Cl − from catalysts prepared using chloride precursors can contaminate the Pt surface [3,5–9] . The adsorption of halide ions on Pt surface increases in the order of F − < Cl − < Br − < I − [4,10,11] . Platinum single-crystal studies have revealed the strong adsorption of chloride ions to the Pt crystal surface planes, thus hindering the oxygen reduction reaction (ORR), especially in electrolytes without strongly adsorbing anions such as HClO 4 [12–14] . Studies have shown that the adsorption of anions on Pt surfaces occurs even at impurity concentrations of less than 10 −6 M [10,11,15] . Schmidt et al. reported an increased H 2 O 2 production with the addition of chloride ions, which could severely degrade the membrane [6] . Yadav et al. found that the dissolution of Pt under potential cycling depends on Cl − concentration [16] . In general, the loss of active Pt surface area for the ORR, a change in the reaction pathway, enhanced H 2 O 2 formation and an enhanced Pt dissolution are some of the major known impacts of chloride ions in PEMFC. To study the kinetics of the catalysts under different conditions, electrochemical experiments with a liquid electrolyte are frequently used to model the real systems. Commercially available reference electrodes, which are invariably a part of these electrochemical cells, often contain chloride-bearing species. These anions could leak into the bulk electrolyte and interfere with the working electrode depending on the operating temperature, working potential and ion concentration. Platinum alloyed with Co, Ni, Cu and other transition metals are generally found to have enhanced ORR activity over pure Pt/C [17–21] . It has been proposed that this enhancement is due to the formation, upon leaching, of alloy particles with a Pt-skin or Pt-shell, with distinctly different electronic properties compared to those of pure Pt [22–27] . Strasser et al. [26] found that the Pt–Pt interatomic distance in the Pt-rich shell on top of a Pt–Cu alloy core is shorter than one would expect based on the composition of this shell, leading to a compressive strain that leads to an enhanced ORR activity. The formation of Pt–OH intermediates, which are considered to block the active Pt site for ORR, is generally found to be delayed in Pt alloys, thus enhancing the ORR activity [28] . In this work, we will show that chloride ions have a large negative impact on the ORR activity of both Pt and PtNi alloys and at the same time accelerate the dissolution of Ni from PtNi alloys. Electrodeposited Pt, Pt 75 Ni 25 and Pt 10 Ni 90 (atomic ratios) were exposed to electrolytes containing chloride at various concentrations under potential cycling. EDS, ICP and XPS were employed to monitor the changes in the Pt:Ni atom ratio in the catalyst layer and in the electrolyte. As the anion impurities from standard reference electrodes such as saturated calomel electrode (SCE) or Ag/AgCl electrode could interfere with the electrochemical measurements and affect the Pt ORR activity [29] from a practical point of view, the consequence of chloride ion leakage from the reference electrode is also investigated. 2 Experimental PtNi alloys were electrochemically deposited from a solution containing 1.5 g l −1 H 2 PtCl 6 ·6H 2 O (Merck), 25 g l −1 NiCl 2 ·6H 2 O (Merck) and 30 g l −1 NaCl (Merck) at pH 2.5 on a finely polished Au rotating disk electrode (RDE). The catalysts were deposited galvanostatically during a fixed time of 550 s. The current of 300 and 900 μA cm −2 was applied respectively to deposit Pt 75 Ni 25 and Pt 10 Ni 90 . For comparison, pure Pt was deposited at 300 μA cm −2 for 500 s from a solution of 1.5 g l −1 H 2 PtCl 6 ·6H 2 O (Merck) at pH 2.5. Prior to deposition, the Au RDE was electrochemically cleaned in 0.5 M H 2 SO 4 (Merck) by potential scanning between 0 V and 1.7 V until a stable cyclic voltammogram (CV) was obtained. The electrochemical cell was flushed with Ar for about 45 min before the catalyst deposition. As the catalyst precursors contain chloride ions, as a precautionary step, the electrode was washed with copious amount of deionized water to remove the likely inclusion of chloride in the catalyst layer. 2.1 Electrochemical characterization The electrolytes with Cl − concentrations of 10 −3 M and 10 −4 M in 0.5 M HClO 4 were prepared from concentrated HCl (32%) and HClO 4 (65%) (both from Sigma–Aldrich) by adding the required amount of HCl to 0.5 M HClO 4 . For all the electrodes (Pt, Pt 75 Ni 25 and Pt 10 Ni 90 ), the potential was scanned between 20 mV and 1.3 V in blank 0.5 M HClO 4 , 10 −3 M HCl in 0.5 M HClO 4 and 10 −4 M HCl in 0.5 M HClO 4 separately at room temperature under argon atmosphere. A three-compartment electrochemical cell was used with the working electrode and the reference electrode compartments separated by a glass frit. As the current work deals with the effect of Cl − impurities, care was taken not to use calomel and other types of reference electrodes that can leak chloride ions. Instead, a reversible hydrogen electrode (RHE) was constructed from a Pt wire that was kept under a saturated hydrogen atmosphere. A platinized platinum flag was used as a counter electrode. The electrodes were initially scanned for 15 CVs to ensure a stable surface. The electrodes were again scanned for 1000 potential cycles to validate their electrochemical stability and modification in their ORR activity under Cl − contamination. Hence, the ORR activity of the alloys at room temperature was determined by recording the polarization curves between 0.2 V and 1.1 V at a rotation speed of 1000 rpm under oxygen-saturated electrolyte after the completion of 15 and 1000 CVs respectively. 2.2 Elemental analysis Energy dispersive X-ray spectroscopy (EDS) was carried out for all the fresh alloys using a Quanta 3D FEG instrument (FEI Company) to check the Pt:Ni atom ratios initially, so as to tune the deposition conditions to the desired elemental ratio. Various spots were selected on the catalyst layer and the concomitant atom% of Ni and Pt were averaged. The change in the Pt:Ni ratio for the alloys after 15 and 1000 potential cycles was also monitored. Likewise inductively coupled plasma (ICP) analysis was performed on the electrolyte to deduce the amount of Pt and Ni dissolved from the alloys during the initial dealloying in both blank HClO 4 and 10 −4 M HCl added HClO 4 . Surface compositions of selected samples were analyzed using a Kratos AXIS Ultra X-ray photoelectron spectrometer (XPS), equipped with a monochromatic Al Kα X-ray source and a delay-line detector (DLD). Spectra were obtained using the aluminum anode (Al KR) 1486.6 eV operating at 150 W, with survey scans at a constant pass energy of 160 eV and with region scans at a constant pass energy of 40 eV. Depth profiles were obtained by sputtering, while rotating the sample, using an Argon pressure of 3 × 10 −8 mbar Argon, while the emission current was set to 15 mA with beam energy of 4 kV. Moreover, the presence of Cl − on the Pt surface before and after exposing to chloride environment was also evaluated using XPS. The electrodes, after exposure to 15 potential scans, were held at 0.95 V for a few seconds to prevent any adsorbed Cl − to escape from the surface. Then the electrode was carefully removed form the electrochemical cell with the potential of 0.95 V still applied. The electrode was cleaned gently with deionized water and then transferred quickly to the vacuum chamber for XPS measurements. 2.3 Effect of Cl − -containing reference electrode To substantiate the influence of chloride ions leaked from a Cl − -containing reference electrode, a few experiments were conducted on PtNi alloys at room temperature and at 80 °C. A commercially available reference electrode (Red Rod Technology from Radiometer Analytical) saturated with KCl and stable in the acidic environment up to 100 °C was used for this purpose. The reference electrode used has a double junction and was placed in a separate compartment in the electrochemical cell to minimize Cl − contamination. The resultant CVs and the spectroscopic results were compared to those of the above-described experiments. 3 Results and discussion 3.1 Electrochemical behavior in blank 0.5 M HClO 4 The hydrogen adsorption/desorption profiles of the PtNi alloys after 15 CVs were clearly different from that of pure Pt ( Fig. 1 a ), suggesting that alloying Ni to Pt influences the adsorption of hydrogen on Pt. Note that, contrary to what the literature leads one to expect (see Section 1 ), there is no difference in the Pt–OH onset potential between Pt and its Ni alloys. This could be a particle-size effect: the Pt electrodeposit has somewhat larger crystallites (4.5 ± 1.2 nm) than the alloys (3.4 ± 1.3 nm, and 3.6 ± 0.9 nm, respectively, for Pt 75 Ni 25 and Pt 10 Ni 90 as determined by TEM [30] ). Thus, the cathodic shift in the Pt–OH onset potential to be expected for smaller particles [31] could be compensated for by the alloy effect. The ORR polarization curves of the catalysts after 15 CVs, normalized to the geometric surface area (0.2826 cm 2 ), are shown in Fig. 2 a . It has to be noted, however, that the electrodeposits are quite porous, so that the measured (kinetic) current has to be normalized to the electrochemical active Pt surface area (ECSA) to arrive at the specific ORR activity. ECSA values were calculated by integrating the area under the H upd region of the CVs and they vary between 3 cm 2 and 6.5 cm 2 for the present catalysts. The thus obtained values for the specific ORR activities are collected in Table 1 . It is seen that at this stage the PtNi catalysts are 3–4 times more active than their Pt-only counterpart. Again, this is in contrast to the literature position that Pt alloys are better ORR catalysts than Pt because of the Pt–OH onset potential shifting to higher potentials, which is clearly not the case here. We surmise that subsurface Ni here accelerates the rate-determining step in the ORR, rather than freeing up more sites to perform the reaction on (see further below). The cyclic voltammograms of the present catalysts after 1000 cycles are presented in Fig. 1 b. Clearly, the leaching of Ni has now progressed to a stage that the hydrogen adsorption/desorption profiles of Pt and of PtNi are quite similar. However, in the Pt-oxide region small differences are still observed. In particular, the Pt–OH onset potential of Pt 10 Ni 90 is somewhat cathodic to that of Pt itself. This cannot now be a particle size effect, as the aged Pt and Pt 10 Ni 90 electrodeposits have crystallites of approximately the same size (5.3 ± 2.2 and 5.1 ± 2.3 nm, respectively; for aged Pt 75 Ni 25 a value of 4.4 ± 2.3 nm was found [30] ). The ORR polarization curves after 1000 CVs are shown in Fig. 2 b. The derived values of the specific ORR activities are given in Table 1 . It is seen that both Pt and its Ni alloys have deactivated to a considerable extent. For Pt this is not so usual, and it is presumably due to a change in morphology. Comparison of Fig. 1 a and b shows that extensive potential cycling has a distinct influence on the structure of the platinum surface. The sharp weakly adsorbed hydrogen oxidation peak around 0.15 V, present in the fresh platinum, becomes much smaller after 1000 CVs. This sharp peak can be assigned to Pt (1 1 0) [32] . At the same time, a peak at the cathodic limit of the CV scan, which is generally attributed to the oxidation of adsorbed molecular hydrogen, becomes apparent in the platinum electrode after 1000 CVs. Such peak is visible in Pt (1 0 0) single crystal electrodes as well. When looking closely to the ORR sweep of the platinum electrode after 1000 CV scans in Fig. 2 b, and comparing them to those in single crystal electrodes as published in [33] , the observed ORR does well correspond to that on Pt (1 0 0). From both [32,33] it is known that the ORR activity on Pt (1 0 0) is far lower than that on Pt (1 1 1) and Pt (1 1 0). The observed change in the hydrogen adsorption profile, the profile of the ORR and the decreased activity match indicate a structural change upon extensive potential cycling. As to the deactivation of the PtNi electrodeposits, they also may have suffered from some morphology change, but this is not so readily apparent from the CVs after 15 and 1000 CVs, and the main cause for the deactivation will have been extensive Ni leaching. Still, they are about twice as active as Pt, after 1000CVs, and again, this is not due to an anodic shift of the Pt–OH onset potential. Thus, the effect of Ni in the present case appears to remain an effect on the ORR itself, rather than on the availability of Pt active sites. It is noted that similar results have been obtained for PtCo and PtCu electrodeposits [30] . 3.2 Electrochemical behavior in HCl-containing 0.5 M HClO 4 Addition of a small amount of HCl (10 −4 M) to the electrolyte leads to a substantial change in the hydrogen adsorption/desorption behavior of Pt. The CV after 15 cycles is shown in Fig. 3 a . The emergence of two sharp, highly reversible peaks instead of one is commonly observed for Pt in the presence of strongly adsorbing anions [10] . For the alloys, such a distinct H upd region in the CV profiles does not emerge ( Fig. 3 a), indicating the continued presence of subsurface Ni, despite the strong Ni leaching in Cl-containing electrolyte (see below, Table 2 ). On the other hand, the shrinkage of the H upd region and the increase in the double-layer region that is a hallmark of Pt under chloride-ion adsorption [10] was observed for both Pt and the Pt alloys with Pt showing the largest effect. The formation of the oxygen layer begins at the lowest potential for Pt 10 Ni 90 , followed by Pt 75 Ni 25 and Pt itself. This implies that the suppression of Pt–OH formation by adsorbed chloride increases in the same sequence. We believe that this effect is mainly due to subsurface Ni influencing the subtle balance in the competition between OH ad and Cl ad for the Pt surface [14] , with Ni leading to a relative favoring of OH over Cl. An additional effect could be the formation of NiCl x species in solution, which would lower the effective Cl − concentration, and, hence, the Cl surface coverage. That such an effect may play a role is shown in Fig. 3 c: upon changing the Ni-containing electrolyte for a fresh one, an anodic shift of the Pt–OH onset potential is observed. Addition of a larger amount of HCl (10 −3 M) to the electrolyte leads to an, on the face of it, simpler picture (see Fig. 4 a ). The H upd region still shows a difference between Pt and its Ni alloys, indicating that some subsurface Ni still survives after 15 cycles in this medium, but the Cl − concentration is now so large that Cl ad dominates over OH ad to quite high potentials, thus almost obliterating the differences between Pt and PtNi in the oxide region. Turning now to the ORR activity of the stabilized electrodes (after 15 CVs), a comparison between Figs. 2a, 5a, and 6 , already show, as expected, the enormous decrease in performance in the presence of chloride ions, due to their blocking of active sites [14] . The various values of the specific activity are collected in Table 1 . Note that the potentials at which these values are quoted are different for the different chloride concentrations. From 5a and 6 , in the presence of chloride, the hysteresis in ORR between anodic and cathodic sweeps tends to decrease considerably. In the absence of chloride ( Fig. 2 a), the hysteresis in the ORR sweeps is caused by strongly adsorbed OH species, which themselves show irreversible adsorption, i.e., the adsorption takes place at a higher potential than desorption. In the anodic scan, the potential range in which the surface is devoid of strongly adsorbed OH extends to higher potentials, and results in a higher ORR activity. In the presence of chloride, its adsorption strongly inhibits the formation of adsorbed hydroxyl ions (as discussed above). While the irreversibility of the adsorption of OH species is associated with the formation of platinum oxide at higher potential, the nature of the adsorbed chloride does not change with potential, and does not show any hysteresis effect. Combining both effects, in the presence of chloride the effect of its adsorption dominates, leading to the virtual absence of hysteresis in the ORR activity. From Table 1 it can be inferred that in the 10 −4 M HCl case the advantage in specific ORR activity of PtNi over Pt has been reduced to about a factor of 1.5. That is, although ( Fig. 3 a) the respective electrodes differ in their affinity of Cl and OH, the combined affect of these two adsorbates almost cancels out the ORR performance difference noted in Cl-free electrolyte. In the 10 −3 M HCl case the differences in specific activity have become even smaller, as Cl adsorption dominates. After 1000 CVs in the 10 −4 M HCl containing electrolyte, the H upd signatures of Pt and PtNi have become much more similar than before the durability test ( Fig. 3 b). As observed in the Cl-free case, the H upd region of the Pt CV has become much more (1 0 0)-like. The hydrogen ad- and desorption profiles of Pt 75 Ni 25 follow those of Pt closely, indicating that very little Ni remains in subsurface positions, while the somewhat more important deviations observed for Pt 10 Ni 90 suggest the retention of more subsurface Ni in this case. For all three electrodes the Pt–OH onset potential has shifted anodically, indicating that the adsorption of chloride ions has become more dominant than after 15 CVs, and this is in agreement with the increased (1 0 0) character of the Pt surface [14] . A small additional effect here may be an increased Cl/electrode surface area ratio, since the ECSA decreases by about 50% during the cycling. Nevertheless, the Pt 10 Ni 90 surface retains some of its higher oxophilicity as compared to the other two surfaces, and this must, at least partly, be due to the continued presence of some subsurface Ni. Another part may be, as noted above, the effective decrease of the concentration of free chloride ions due to the formation of NiCl x species in solution. The specific ORR activities of the electrodes after 1000 CVs in the presence of 10 −4 M chloride are collected in Table 1 . As before, it is seen that Pt has deactivated to a considerable extent, and this is again ascribed to its surface having become more predominantly Pt (1 0 0). While Pt 75 Ni 25 is about two times as active now as Pt itself, Pt 10 Ni 90 has lost much less of its initial activity, ending up as about six times as active as Pt. So, the superior ORR activity of aged Pt 10 Ni 90 , as compared with that of aged Pt, continues to assert itself (cf. Table 1 for the Cl-free case), and this must, at least partly, be, it is surmised, an effect of the presence of subsurface Ni. The durability of the electrodes in the 10 −3 M chloride case could not be studied, since they were already completely dissolved after some 300 cycles, exposing the Au substrate ( Fig. 4 b). This evidences that chloride ions enhance the dissolution of Pt. 3.3 PtNi composition variation It is well known that the electrochemical potential cycling under the relevant potential range for PEMFC dissolves the less noble metal of the alloy, while enriching the surface layers with the more noble metal. From Table 2 it becomes apparent that the dissolution rate of Ni from the alloys in HClO 4 solution is significantly lower than in the chloride-containing electrolyte. In Pt 75 Ni 25 and Pt 10 Ni 90 about 17 and 58 atom % Ni remained, respectively, after 15 CVs in chloride free HClO 4 . The same experiment in the presence of Cl − gave values of 14 and 22 atom % Ni after the same number of CVs. The presence of Cl − preferentially increases the dissolution rate of Ni from the alloys during the initial potential cycling, irrespective of the concentration of HCl (10 −3 or 10 −4 M). The rate of Ni dissolution decreases during subsequent potential cycling and this is understood by the formation of a Pt skin. The amount of Ni present in the alloys after 1000 CVs was fairly similar irrespective of the initial Pt:Ni concentration and the constituents in the electrolyte. Still, there are indications (e.g., Fig. 3 b) that a bit more subsurface Ni is retained in Pt 10 Ni 90 than in Pt 75 Ni 25 . 3.4 ICP analysis From EDS analysis, it was found that the Ni dissolution takes place predominantly during the initial stages of potential cycling. So, to complement the above findings, the amount of Pt and Ni dissolved in the electrolyte was analyzed at the end of 15 CVs by ICP elemental analysis. In all the cases, be it in blank HClO 4 or in the presence of Cl − , the exact quantity of Pt could not be established. The amount of Pt found in the electrolyte after 15 CVs was below the detection limit of the ICP (0.002 mg l −1 ). The very low concentrations of Pt in the electrolyte might be due the redeposition of dissolved Pt on the catalyst surface during the subsequent potential scans. However, as understood from the CVs ( Fig. 4 b) the dissolution of Pt was high in chloride-rich electrolyte. On the other hand, the amount of dissolved Ni from the alloys could be determined accurately and the data are given in Table 2 . For Pt 75 Ni 25 , the amount of dissolved Ni was similar in blank HClO 4 as well as HClO 4 with 10 −4 M HCl. For Pt 10 Ni 90 , the difference in the Ni dissolution rate was well recognizable when dealloyed in the presence and absence of Cl − in the electrolyte, that is, the amount of Ni dissolved in the presence of 10 −4 M HCl is more than twice the amount dissolved in blank HClO 4 . 3.5 Impact of chloride ion leakage from KCl-saturated reference electrodes The above results indicate that Cl − contamination in PEMFC even in the range of ppm levels will invariably affect the electrocatalytic activity of Pt and Pt alloys besides enhanced Pt and Ni dissolution. The CV and RDE profiles of PtNi alloys in 10 −4 M HCl suggest a distinct although minimal enhancement in their ORR activity compared to Pt but in higher Cl − concentration the performance of all the catalysts was alike. A comparison of the stable CV of Pt 10 Ni 90 measured using a RHE and the KCl-saturated reference electrode (Red Rod) at room temperature and at 80 °C is shown in Fig. 7 a and b . Features similar to what discussed in the previous sections were noticed for the electrode exposed to the chloride-containing reference electrode. The specific activity shown in Table 1 reveals that the performance of the catalysts measured using the chloride-containing reference electrode is very similar to what was found in 10 −4 M HCl. The Cl − -leakage rate from the reference electrode would be more at higher temperatures and hence a greater effect of Cl − was witnessed for the same alloy at 80 °C. Hence, care must be taken with the choice of reference electrode when studying the alloying effect of Pt electrocatalysts. Even a small leakage of Cl − (<5 ppm) from the reference electrode will drastically increase the non-noble metal dissolution rate and influence the ORR activity. 3.6 Electrode surface analysis by XPS As chloride-based precursors were used for the electrodeposition of alloys, it is crucial to analyze the catalysts layer for the presence of remnant chloride. The EDS and XPS analyzes could not detect any chloride on the bulk and surface of the electrodes and the respective results are shown in Fig. 8 a . The XPS data confirm the presence of adsorbed Cl − on the surface of electrodes exposed to Cl − -containing electrolyte ( Fig. 8 a). The peak at ∼199.0 eV and at ∼208.0 eV belong to Cl 2p position, while the former peak represents Cl in chloride ion while the latter corresponds to Cl in ClO 4 − . This also suggests the adsorption of weakly adsorbing perchlorate ions onto the platinum surface in addition to strongly adsorbing Cl ions at more anodic potentials (in this case a standby potential of 0.95 V is applied). The Pt:Ni surface compositions of the fresh alloys and potentially cycled (15 CV) in blank, chloride-containing electrolyte and blank electrolyte with KCl saturated reference electrode at 80 °C are shown in Table 2 and Fig. 8 b. While the surface of Pt 75 Ni 25 is depleted of Ni atoms upon exposing to the 10 −4 M HCl-containing electrolyte, Pt 10 Ni 90 retained about 10 atom% Ni under the same conditions. However, the HCl concentration of 10 −3 M in the electrolyte completely leaches out the Ni atoms from the surface of both the alloys. The absence of Ni atoms on the surface of the electrodes that were open to KCl saturated reference electrode could also be partially due, of course, to the high operating temperature (80 °C) besides the chloride-ion effect. 4 Conclusion In this work we show that the presence of even small amounts of Cl − in the electrolyte will rapidly increase the dissolution rate of Ni from PtNi alloys. It is found that the dissolution takes place predominantly during the first few electrochemical cycles. Thereafter, as follows from the elemental compositions, the electrode surface is enriched with Pt (Pt-skin) that prevents further Ni dissolution from the electrode bulk. At higher chloride concentrations (10 −3 M HCl), however, the complete electrodeposit dissolves upon cycling a few 100 times. The ORR activity of Pt and PtNi alloys is severely impacted by the presence of chloride in the electrolyte, decreasing about two orders of magnitude at 10 −4 M Cl − . After 1000 cycles the ORR activity of PtNi is still somewhat higher than that Pt, indicating that, especially in the case of Pt 10 Ni 90 , some Ni is retained in subsurface positions, but it is clear that the presence of Ni does not mitigate the effect of Cl − on electrode performance. In all, it can be concluded that even minimal amounts of Cl − are deleterious for the ORR on Pt and PtNi and hence needs to be avoided completely from the fuel cell systems. Acknowledgements This work was part of the Dutch EOSLT Consortium PEMFC, Contract Nos. EOSLT 06005 and EOSLT 07005, supported by the Ministry of Economic Affairs . Ad Wonders, Ajin Cheruvathur and Adelheid Elemans-Mehring (all from Eindhoven University of Technology) are gratefully acknowledged for their technical support. References [1] Y. Nagahara S. Sugawara K. Shinohara J. Power Sources 182 2008 422 [2] W. Schmittinger A. Vahidi J. Power Sources 180 2008 1 [3] A.J. Steinbach C.V. Hamilton Jr. M.K. Debe ECS Trans. 11 2007 889 [4] R. Mohtadi W.K. Lee J.W. Van Zee J. Power Sources 138 2004 216 [5] A.B. Ofstad M.S. Thomassen J.L. Gomez de la Fuente F. Seland S. Moller-Holst S. Sunde J. Electrochem. Soc. 157 2010 B621 [6] T.J. Schmidt U.A. Paulus H.A. Gasteiger R.J. Behm J. Electroanal. Chem. 508 2001 41 [7] M.S. Mikkola T. Rockward F.A. Uribe B.S. Pivovar Fuel Cells 7 2007 153 [8] F.J. Luczak, US. Patent 4447506, 1984. [9] M. Watanabe M. Uchida S. Motoo J. Electroanal. Chem. 229 1987 395 [10] M.W. Breiter Electrochim. Acta 8 1963 925 [11] B.E. Conway D.M. Novak J. Mozota J. Electrochem. Soc. 128 1981 C116 [12] V. Stamenkovic N.M. Markovic P.N. Ross J. Electroanal. Chem. 500 2001 44 [13] N.M. Markovic H.A. Gasteiger B.N. Grgur P.N. Ross J. Electroanal. Chem. 467 1999 157 [14] N.M. Markovic P.N. Ross Surf. Sci. Rep. 45 2002 121 [15] A. Zolfaghari B.E. Conway G. Jerkiewicz Electrochim. Acta 47 2002 1173 [16] A.P. Yadav A. Nishikata T. Tsuru Electrochim. Acta 52 2007 7444 [17] H.A. Gasteiger S.S. Kocha B. Sompalli F.T. Wagner Appl. Catal. B: Environ. 56 2005 9 [18] K. Jayasayee V.A. Dam T. Verhoeven S. Celebi F.A. de Bruijn J. Phys. Chem. C 113 2009 20371 [19] S. Mukerjee S. Srinivasan J. Electroanal. Chem. 357 1993 201 [20] U.A. Paulus A. Wokaun G.G. Scherer T.J. Schmidt V. Stamenkovic V. Radmilovic N.M. Markovic P.N. Ross J. Phys. Chem. B 106 2002 4181 [21] R. Srivastava P. Mani N. Hahn P. Strasser Angew. Chem. Int. Ed. 46 2007 8988 [22] R.R. Adzic J. Zhang K. Sasaki M.B. Vukmirovic M. Shao J.X. Wang A.U. Nilekar M. Mavrikakis J.A. Valerio F. Uribe Top. Catal. 46 2007 249 [23] K. Sasaki Y. Mo J.X. Wang M. Balasubramanian F. Uribe J. Mcbreen R.R. Adzic Electrochim. Acta 48 2003 3841 [24] V. Stamenkovic T.J. Schmidt P.N. Ross N.M. Markovic J. Phys. Chem. B 106 2002 11970 [25] V. Stamenkovic T.J. Schmidt P.N. Ross N.M. Markovic J. Electroanal. Chem. 554 2003 191 [26] P. Strasser S. Koh T. Anniyev J. Greeley K. More C.F. Yu Z.C. Liu S. Kaya D. Nordlund H. Ogasawara M.F. Toney A. Nilsson Nat. Chem. 2 2010 454 [27] T. Toda H. Igarashi H. Uchida M. Watanabe J. Electrochem. Soc. 146 1999 3750 [28] V. Stamenkovic B.S. Mun K.J.J. Mayrhofer P.N. Ross N.M. Markovic J. Rossmeisl J. Greeley J.K. Norskov Angew. Chem. Int. Ed. 45 2006 2897 [29] K.J.J. Mayrhofer S.J. Ashton J. Kreuzer M. Arenz Int. J. Electrochem. Sci. 4 2009 1 [30] K. Jayasayee, PhD Thesis, Eindhoven University of Technology, The Netherlands, 2011. [31] K.J.J. Mayrhofer B.B. Blizanac M. Arenz V.R. Stamenkovic P.N. Ross N.M. Markovic J. Phys. Chem. B 109 2005 14433 [32] N.M. Markovic R.R. Adzic B.D. Cahan E.B. Yeager J. Electroanal. Chem. 377 1994 249 [33] V. Komanicky H. Iddir K.C. Chang A. Menzel G. Karapetrov D. Hennessy P. Zapol H. You J. Am. Chem. Soc. 131 2009 5732
更多查看译文
关键词
PtNi alloys,Chloride ion,PEMFC contamination,ORR activity,Fuel cells
AI 理解论文
溯源树
样例
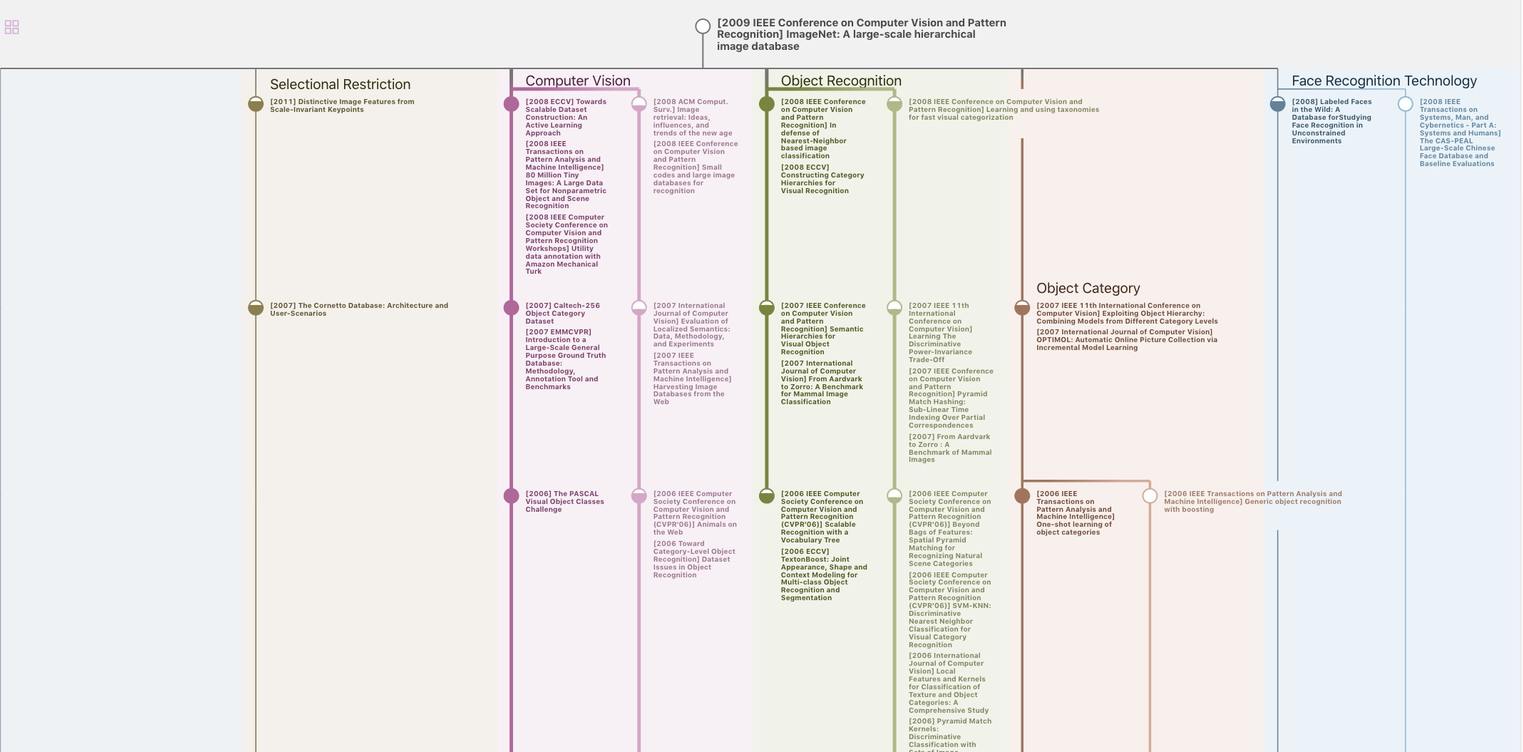
生成溯源树,研究论文发展脉络
Chat Paper
正在生成论文摘要