Injectable gel scaffold based on biopolymer microspheres via an enzymatic reaction.
ADVANCED HEALTHCARE MATERIALS(2014)
摘要
Injectable gel scaffolds are produced via an enzymatic reaction and extra electrostatic interactions between enzymatic peptide-functionalized heparin microspheres and a chitosan liquid phase. As a result of the controllability of this approach, the gel scaffolds with double-network structures can render the formulation of a therapeutically effective platform for tissue engineering. Macromolecular microspheres have become an important structure in polymeric materials.1 Microspheres made from biopolymers, such as chitosan, alginate, hyaluronan, and gelatin, are widely used in biomedical applications as drug carriers, cell scaffolds, and artificial tissues. Recently, an assembly of biopolymer microspheres has become of increasing interest in the production of biofunctional gel scaffolds for drug or cell delivery applications.2 The development of microsphere-based gel scaffolds with high bioactivity and suitable mechanical performance would greatly broaden the application for soft tissue and cartilage regeneration. However, it is difficult to form bulk hydrogels using biopolymer microspheres, and, when formed, the microsphere gels do not exhibit suitable mechanical strength or bioactive property. Many efforts have focused on interparticle interactions for assembly of microspheres, including electrostatic forces,3 magnetic forces,4 hydrophobic interactions,5 and steric hindrance,6 but the robustness and bioactivity of formed gels still remain unsatisfactory. Although some microspheres based on synthetic polymers are quite successful for bulk scaffolds, the biomedical applications of these scaffolds are strongly limited by the intrinsic inability to translate cell growth factors (GFs) into tissue engineering applications.7 There is a clinical need for the development of bioactive microsphere-based gel scaffold that approximates the delivery of GFs for tissue regeneration. Recently, the double-network (DN) principle provides a general approach for developing robust hydrogels for biomedical applications. For example, Gong, Khademhosseini, and colleagues have reported a new method of obtaining strong and tough hydrogels by making DN materials with a high molar ratio of the second network to the first network.8 Further research into these materials as tissue engineering scaffolds determined that these DN hydrogels weakened when they were prepared in cell-compatible solutions, and the encapsulated cells did not function as well as in single network hydrogels. Following this approach, the microgel-reinforced strategy was used by Khademhosseini and co-workers to make hydrogels by embedding stiff microgels into a soft and ductile matrix.9 These hydrogels exhibited significantly higher strength than single network hydrogels with no microgels, and comparable strength to DN hydrogels. Motivated by the need to develop better hydrogel systems in terms of both mechanical strength and biological properties, we hypothesize that the microphase-segregated DN concept could be applicable for developing injectable microsphere-based gel scaffolds with biological functionalities. It consists of two networks: a densely cross-linked biopolymer microspheres network (skeleton) and a sparsely cross-linked ductile biopolymer network. One can expected two advantages of this microsphere-based DN gel. First, microspheres incorporated at higher composition ratio can potentially increase strength of the DN gel due to their higher stiffness. Second, because GFs were encapsulated not in the ductile network but in microspheres, the GFs were expected to function better in this microsphere-based DN gel than in normal DN hydrogels. Importantly, unlike most microspheres that are chemically inert, our biopolymer microspheres would have residual functionalities that allow for further bioconjugation of a ductile network. Our strategy is to use heparin as the microspheres substrate and chitosan as the ductile network, respectively. In this system, heparin microspheres with higher composition ratio can potentially increase strength of the DN gel, while chitosan solution as a liquid phase can provide the injection. Heparin and chitosan are proteoglycans or glycosaminoglycans (GAGs), as highly charged polyelectrolytes, which could be additionally crosslinked by electrostatic interactions. Heparin is a negatively charged GAG, which is composed of repeated disaccharide units of alternating glucosamine and glucuronic residues heterogeneously modified by carboxyl groups and N- or O-linked sulfate. Most importantly, the ability of heparin to sequester and stabilize GFs due to specific affinity GF-immobilization has been exploited in the production of microspheres that can mediate cell proliferation and differentiation.10 Chitosan is a positively charged polysaccharide composed of repeated glucosamine and N-acetylglucosamine, which also has been widely applied in drug delivery, gene therapy, and tissue engineering.11 Mechanism for the formation of the microspheres, as well as for the formation of a 3D gel scaffold, is proposed in Scheme 1. Our method for preparing heparin microspheres and gel scaffolds relies on in situ cross-linking of heparin with built-in fibrin-mimicking peptide of CTIGEGQQHHLGGAKQAGDV containing Lys and Gln functionalities. This peptide derived from the transglutaminase factor XIIIa (FXIIIa) crosslinking site of fibrin, with an additional N-terminal cysteine residue.[12] The peptides would couple and form covalent isopeptide bridges between Gln and Lys by enzymatic catalysis of the FXIIIa.12 To do this, chemical modifications were employed to selectively introduce complementary reactive peptides to maleimide-functionalized heparin and chitosan via the Michael-type addition, respectively. Subsequently, peptide-functionalized heparin was formed microspheres via the inverse emulsion cross-linking technique upon FXIIIa-catalyzed cross-linking. Based on our previous optimization of the enzymatic reaction, the microspheres with controlled cross-linking degree and particle size were generated when the cross-linking was carried out within the microemulsions. For formation of the microphase-segregated DN structure, the peptide-functionalized chitosan solution was introduced as the second network and mixed with the peptide-functionalized heparin microspheres to form the contrasting topological structure. The macroscopic gel scaffold was assembled with interlocked hierarchical organizations form through simultaneously enzymatic reactions and extra electrostatic interactions between the peptides on microspheres and those in chitosan solution. There are two levels of cross-linking in this gel scaffold: the primary network consisting of cross-linked heparin chains inside each microsphere, and the secondary network interconnecting the microspheres. Concentration of peptide-functionalized heparin has a significant influence on microsphere size (Figure 1a). With 2 wt% of peptide-functionalized heparin, the number-averaged size of microspheres was 0.64 μm. The microspheres showed a slightly larger number-averaged size of 0.78 μm after swelling for 24 h in phosphate buffered saline (PBS) at 4 °C (Figure 1b). Scanning electron microscopy (SEM) was utilized to visualize the morphology of microspheres. As shown in Figure 1c, the prepared microspheres were well-defined spheres with smooth surfaces irrespective of the peptide content in the composition. Generally, fibrin is susceptible to degradation (fibrinolysis) by various proteolytic enzymes, including plasmin and matrix metalloproteases (MMPs).12 Due to its intrinsic protease substrate in this peptide, the peptide-functionalized heparin microspheres, which under in vitro conditions are stable for many weeks, are readily degraded when exposed to active MMP-1. As shown in Figure 1d, the cavities and cracks are seen on the surface of lyophilized microspheres after incubation with 40 × 10−9 m MMP-1 at 37 °C for 24 h, clearly indicating the accessibility of enzymatic linkages on the microspheres. Therefore, one can conclude that exposure of the heparin microspheres to MMPs induces microsphere biodegradation and thereby could release GFs into the microenvironment. Composition and gelation characteristics of gel scaffolds are shown in Table 1. Notably, the gel scaffold formed from heparin microspheres is highly elastic networks with the chitosan concentration of 2 wt% and the microsphere/liquid volume ratio of 7:3. On the other hand, upon mixing with the higher microsphere/liquid volume ratio, e.g., 9:1, microspheres did not form macroscopic gels even with prolonged incubation overnight at 37 °C. Higher concentration of peptide-functionalized chitosan also leads to tougher gel scaffolds. These observations suggest that the 7:3 volume ratio of microsphere/liquid and 2 wt% of liquid phase had relatively higher amounts of peptides than other formulations required for a tough gel scaffold. Since the theoretical closest compact factor for spheres is 0.74, implying that at least 26% space is left in the piled microspheres. The formulation of 7:3 volume ratio of microsphere/liquid showed a theoretical space of 30%, which was close to the compact space of 26%. We speculate that this gel scaffold occupied more suitable space to chitosan liquid phase for cross-linking of gel formation than other formulations, for example, 9:1 (10%) and 5:5 (50%) ratios. As a result, this gel scaffold toughened higher because the cross-linking density or stacking density among the microspheres was relatively high comparing other ratios and concentrations. Therefore, this optimal volume ratio of 7:3 and liquid concentration of 2 wt% functionalized chitosan were used for the next study. Gelation rate of gel scaffolds can be controlled by the concentration of FXIIIa, allowing us to fine-tune the kinetics to an application of interest. Upon mixing with FXIIIa from 2 to 10 U mL−1, the microspheres led to self-supporting gel scaffolds within 15 min (Figure 2a). Dynamic time sweep rheological experiment was conducted to monitor scaffold network evolution during the gelation process in situ at 37 °C. Upon 10 U mL−1 FXIIIa, macroscopic gel scaffolds were obtained within less than 5 min at which the elastic modulus (G′) was equal to the storage modulus (G″) (Figure 2b). Such rapid curing kinetics are suitable for in situ tissue engineering applications. The data also indicate a final G’ value of ≈7.27 kPa at time of ≈13 min, signifying a structurally robust network that maintains its 3D shape with loading. Generally, the micro-/nanosphere assembled gels, which were either covalently or physically, are usually very soft with nominal G′ ranging from 10 to 102 Pa.2, 3 Thus, unlike conventional microspheres-based hydrogels, this gel scaffold is much more stiff and strong than biological soft tissues. Such rheological behavior is characteristic of solid-like gel material, hence, it may be suitable for some tissue regeneration applications, e.g., adipose muscle and cartilage. Fourier transformed infrared (FTIR) spectrum of gel scaffold was measured to confirm the chemical composition (Figure 2c). The functionalized chitosan showed the basic characteristic peak at 1598 cm−1 (NH bend) in the spectrum, which suggests that it was involved in gel formation. This viscoelasticity behavior makes the gel scaffolds suitable for extrusion and injection by syringes. As is clearly illustrated in Figure 2d, homogeneous gel threads were formed upon injection through a conventional medical syringe. SEM images revealed the microstructure of lyophilized gel scaffold. As shown in Figure 2e, intersphere distances were considerably close and showed a clear interconnection between heparin microspheres. By the additional liquid phase of peptide-functionalized chitosan, the gel scaffold showed a compact packing of microspheres via the enzyme-catalyzed reaction. The lyophilized peptide-functionalized chitosan was also found between microspheres (Figure 2f), which might contribute to increased cross-linking of the gel scaffold via extra electrostatic interactions. Figure 2g displayed the microstructure of lyophilized control sample without peptide-functionalized chitosan. Comparing to the gel scaffold with functionalized chitosan, the control sample showed a differently smooth morphology. Therefore, we can speculate that the peptide-functionalized chitosan in the liquid phase could couple microspheres as bridges. We speculate that gel scaffolds were also susceptible to biodegradation by proteolytic enzymes of MMPs. This degradation is critical during tissue regeneration, as it enables cells to invade the matrix and is also the basis for subsequent remodeling and morphogenesis to form functional tissue. Therefore, the weight loss and MMP-1 resistance of gel scaffolds were monitored as a function of incubation time in PBS at 37 °C. The concentration of MMP-1 has a significant influence on the weight loss behavior of gel scaffolds. Higher concentration of MMP-1 resulted in faster weight loss of gel scaffolds, which completely dismissed within 4 d in 40 × 10−9 m MMP-1 (Figure 3a), whereas the samples could last more than 3 weeks in PBS at 37 °C without MMP-1. It is worth mentioning that gel scaffolds made by the lower concentrations of peptide-functionalized chitosan (0.5 or 1 wt%) dismissed readily overnight under 40 × 10−9 m MMP-1. Swelling properties of gel scaffolds are important for substance exchange during tissue regeneration.13 Figure 3b indicates equilibrium swelling ratios of the gel scaffold determined in PBS at 37 °C. It is noticeable that the gel scaffold showed initially higher swelling ratio than the control heparin microspheres, which were 1.8 and 0.7, respectively. Swelling ratio of the gel scaffold showed continuously increase up to 14 d during incubation, and the values significantly increased comparing to the control of microspheres (p < 0.05). These observations indicate that the gel scaffold with special DN structures has a higher capacity of water binding than its corresponding particulates. We are interested in utilizing this gel scaffold for treatment of GFs delivery and tissue engineering. Controlled release of GFs can be achieved through their anchorage at predetermined locales of the heparin microsphere-based gel scaffold system. To demonstrate the utility and versatility of the gel scaffolds for GFs release, the bone morphogenetic protein 2 (BMP-2) was employed as the model GFs for affinity delivery, which was verified by the enzyme-linked immunosorbent assay (ELISA). For this purpose, BMP-2-loaded heparin microspheres were prepared using the same conditions in presence of BMP-2, which showed an encapsulation of 27.7 ng of BMP-2 per mg of microspheres, giving a entrapment of 68.5%. As BMP-2 was affinitively encapsulated in heparin microspheres, the results suggest the gel scaffold that was capable of controlled delivery (Figure 3c). Strikingly, over a initial period of 24 h, less than 2.5% of encapsuled BMP-2 was released from the gel scaffold, which showed significantly slower rate compared to the heparin microspheres (p < 0.05). It should be mentioned that the degradation of gel scaffold and microspheres dominated the BMP-2 release in our case. BMP-2 slowly diffused out of the gel scaffold and microspheres, indicating that the heparin is sufficient to support prolonged entrapment. We also checked a control in which BMP-2 in solution was subjected to the same treatment conditions to confirm the quantification. It showed that the immunoassay results were not affected by HCl treatment may due to its low concentration. Furthermore, exposure of the gel scaffold to MMP-1 induced network biodegradation and thereby released BMP-2 into the microenvironment (Figure 3d). An increase in release intensity was observed through the action of 40 × 10−9 m MMP-1 added to the system after 24 h, and the gel scaffold rapidly degraded through the protease action within the next 48 h and released the scaffold matrix-bound BMP-2. Hence, this in vitro delivery of BMP-2 from the gel scaffold is supposed to become controlled by cellular stimuli: when cells infiltrate the scaffold matrix they secrete proteases that liberate the GFs from the scaffold localizing the GF response. These results illustrated the potential for multifunctional gel scaffold and suggested opportunities for controlled BMP-2 delivery in soft tissue and cartilage regeneration. Although the feasibilities of enzymatic cross-linking have been proved by several reports, the in vivo FXIIIa usage should be further investigated by well-designed studies. In human, FXIIIa is essential for hemostasis, and its deficiency results in severe bleeding diathesis.14 The main hemostatic function of FXIIIa is the mechanical stabilization of fibrin clot and the protection of newly formed fibrin from the prompt elimination by fibrinolysis. However, FXIIIa also exerts the anti-thrombotic effect by the inhibition of platelet adhesion to fibrin, which might be involved in limiting the thrombus size by down-regulating the accumulation of platelets on fibrin. Although the effects of FXIIIa on final fibrin clot structures have been examined, the association of FXIIIa level with clinical thrombotic risk was investigated only in a few studies from which no clear conclusion could be drawn. Further studies are required to explore gender- or age-specific differences in the relationship of FXIIIa level and the thrombotic risk. In conclusion, we have developed a heparin microspheres-based gel scaffold with tunable properties for use in GFs delivery. Heparin microspheres were prepared by cross-linking heparin derivative via enzymatic reaction within the inverse emulsion droplets. The microspheres exhibited residual functional peptides that could be used as reactive handles for subsequently enzymatic conjugation of chitosan derivative. The nature of oppositely charged proteoglycans also allowed for simultaneous cross-linking of the microspheres via extra electrostatic interactions, giving rise to doubly cross-linked gel scaffold with tunable viscoelasticity. In vitro degradation studies indicate that the gel scaffold exhibited proteolytically sensitive, and BMP-2 could release controlled upon cell-derived proteolytic degradation of the gel scaffold. We anticipate that this microsphere-based gel scaffold can render the formulation of a therapeutically effective platform for soft tissue and cartilage regeneration. Materials: Heparin (sodium salt, Mw 18 kDa), 1-ethyl-3-(3-dimethylaminopropyl) carbodiimide hydrochloride (EDC·HCl), N-(2-aminoethyl) maleimide trifluoroacetate salt (AEM), 4-maleimidobutyric acid N-hydroxysuccinimide ester (GMBS), 1-hydroxybenzotriazole hydrate (HOBT), thrombin, and 2-(N-morpholino) ethanesulfonic acid (MES) were purchased from Sigma-Aldrich. O-Carboxymethyl chitosan (deacetylation degree 90%, Mn 2 × 105) was purchased from G.T.C. Bio. Corp. (Qingdao, China). Active matrix metalloproteases (MMP-1) was purchased from Sino Biological Inc. (Beijing, China). Recombinant human BMP-2 (rhBMP-2) and the BMP-2 Quantikine ELISA Kit were obtained from R&D Systems (Minneapolis, USA). All other chemicals were used as received. Synthesis of Maleimide-Functionalized Heparin: The synthesis of maleimide-functionalized heparin was performed as previously described with slight medication.15 Briefly, 300 mg heparin (0.02 mmol) was dissolved with 103 mg HOBt (0.67 mmol), 103 mg AEM (0.67 mmol), and 103 mg EDC·HCl (0.54 mmol) in 50 mL of 0.1 m MES (pH 5.5–6.0). The reaction proceeded overnight at room temperature with stirring. The product was purified by dialysis (MWCO 3500) against 1 m NaCl solution and then subsequently against ultrapure water for 3 d. The solution was lyophilized to give a slightly yellowish solid and characterized via 1H NMR (Bruker Avance). 1H NMR (400 MHz, D2O, δ): 7.85 (2H, COCHCHCO, m), 3.87–3.46 (4H, CH2CH2NH, m), 5.40–3.40 (72H, heparin, m). Synthesis of Maleimide-Functionalized Chitosan: 200 mg O-Carboxymethyl chitosan was dissolved with 103 mg HOBt (0.67 mmol), 105 mg GMBS (0.66 mmol), and 103 mg EDC·HCl (0.54 mmol) in 100 mL of 0.1 m MES (pH 5.5–6.0). The reaction proceeded overnight at room temperature with stirring. The product was purified by dialysis (MWCO 10 000) against deionized water and lyophilized to give white solid foam (92% yield). 1H NMR was also used to characterize the maleimide functionality of chitosan molecule at ambient temperature using D2O as a solvent. Peptide Synthesis: The 20 mer peptide CTIGEGQQHHLGGAKQAGDV derived from the Factor XIII crosslinking site of fibrin, with an additional N-terminal cysteine residue, was synthesized using standard solid phase methods as previously described.12 The peptide was cleaved with a mixture of 95% trifluoroacetic acid, 2.5% ethanedithiol, and 2.5% H2O for 1.5 h at room temperature, and then the mixture was frozen and lyophilized to a powder. The crude peptide was then analyzed for purity by reverse phase high performance liquid chromatography (RP-HPLC). Conjugation of Peptide to Biopolymers: Peptide was coupled to maleimide-functionalized heparin and chitosan via Michael-type addition, respectively. Peptide was added to maleimide-functionalized heparin and chitosan in 1.2-fold molar excess over maleimide groups in 0.3 m triethanolamine (pH 8.0) at 37 °C for 3 h. The reaction solutions were subsequently dialyzed (MWCO 3500) against ultrapure water for 3 d at 4 °C. After dialysis, the products were lyophilized at −50 °C to obtain white solid foams. 1H NMR spectroscopy results indicated that the peptide functionalities on heparin and chitosan were estimated to be 56.3% and 67.0%, respectively. Preparation of Microspheres: For enzymatic cross-linking, 100 mL of FXIIIa (100 U mL−1) was activated in presence of 2.5 × 10−3 m CaCl2 with 10 mL of thrombin (20 U mL−1) for 30 min at 37 °C. Small aliquots of activated FXIIIa were stored at −20 °C for further use. Heparin-based microspheres were prepared by cross-linking peptide-functionalized heparin in an inverse emulsion via the inverse emulsion cross-linking technique. The inverse emulsion was prepared by homogenizing peptide-functionalized heparin (3 mL, 2 wt% in H2O) in mineral oil (60 mL) containing 0.3 mL of Span 80 for 5 min using a homogenizer. Activated FXIIIa solution was then added to the emulsion with a final concentration of 10 U mL−1 in the aqueous phase, which was further homogenized for 2 min. The aqueous phase was allowed to evaporate overnight at 37 °C with constant stirring. Microspheres were isolated by precipitation in a large excess of isopropyl alcohol followed by centrifugation to remove the oil phase. The resulting microspheres were thoroughly washed with isopropyl alcohol, hexane, and acetone before being dried under vacuum at room temperature. The average diameters of the microspheres were determined using a Malvern Zetasizer Nano ZS instrument at room temperature. Preparation of Microsphere-Based Gel Scaffolds: The obtained heparin-based microspheres were immersed in PBS at 4 °C to allow overnight swelling. The swollen microspheres were centrifuged at 3000 rpm for 10 min to remove the excess water. The particles were then thoroughly mixed with peptide-functionalized chitosan (0.5, 1, and 2 wt% in deionized H2O) and FXIIIa (2, 5, and 10 U mL−1 in chitosan solution) using syringes at 37 °C. FTIR spectrum of gel scaffold was measured to confirm the chemical composition. Lyophilized sample was recorded with FTIR spectrometer (Nicolet Avatar 360, USA) against a blank KBr pellet background. Swelling Ratio: For swelling experiment, gel scaffolds were immersed in PBS at 37 °C for 1 h until equilibrium of swelling had been reached. The swollen scaffolds were removed and immediately weighed (Ws) after the excess of water lying on the surfaces was absorbed with a filter paper. The swollen scaffolds were then lyophilized at −50 °C and weighted (Wd). The swelling ratio was calculated using the equation of SR = (Ws–Wd)/Wd. Enzymatic Degradation: To study the kinetics of proteolysis, gel scaffolds were incubated in PBS at 37 °C containing MMP-1 (10 × 10−9, 20 × 10−9, and 40 × 10−9 m), respectively. Fresh enzyme solution was replaced every day. The time for complete dissolution of scaffolds was noted. The weight of scaffolds was measured at predetermined intervals during incubation. Morphology: The microspheres and gel scaffolds were critical point dried or lyophilized at −50 °C and then gold-coated using a Cressington 108 Auto (Cressington, Watford, UK). The cross-sectional morphologies were viewed using a JSM-6330F SEM (JEOL, Peabody, MA) operated at 10 kV accelerating. Rheological Experiment: Gelation kinetics of the gel scaffolds were evaluated using a commercial rheometer (AR2000, TA Instruments, New Castle, USA) with the parallel plate geometry (25 mm in diameter). The reaction mixture was quickly mixed and poured onto the center of the bottom plate. Standard low viscosity oil was spread at the side of the geometry to prevent water evaporation during the measurements. The evolution of elastic (G′) and storage (G″) moduli at a constant frequency of 0.5 Hz and constant strain of 0.05 was recorded as a function of time. The gelation time, as a kinetic parameter for network formation, was defined as the time of crossover of G′ and G″. GFs Release: Gel scaffold containing BMP-2 was prepared using BMP-2-loaded heparin microspheres. BMP-2-loaded heparin microspheres were prepared using the above same conditions in presence of BMP-2 (2 μg), which was dissolved into heparin solution (3 mL) at 4 °C. For BMP-2 loading capacity measure, microspheres (5 mg) were completely dismissed overnight in a HCl solution (0.02 N). After removal of HCl at 70 °C, the residues were dissolved in water. According to the immunoassay measurement, BMP-2-heparin microspheres showed an encapsulation of 27.7 ng of BMP-2 per mg of microspheres, giving a entrapment of 68.5%. For in vitro release experiment, BMP-2-loaded microspheres (10 mg) and gel scaffolds (200 μL) were suspended in 1 mL of PBS at 37 °C, provided a reservoir into which peptide could be released from the scaffolds complex and subsequently measured during degradation. At predetermined intervals, a 200 μL sample of release medium was extracted from the sample vials and replenished with 200 μL fresh release medium to maintain a constant volume. Samples were centrifuged and supernatants were collected and stored at −80 °C until analysis by the immunoassay measurement. This study is financially supported by National Natural Science Foundation of China (51103071), Natural Science Foundation of Jiangsu Province (BK2011714) and Zijin Intelligent Program of NJUST.
更多查看译文
关键词
biopolymers,cell growth factor,gel scaffolds,microspheres,tissue engineering
AI 理解论文
溯源树
样例
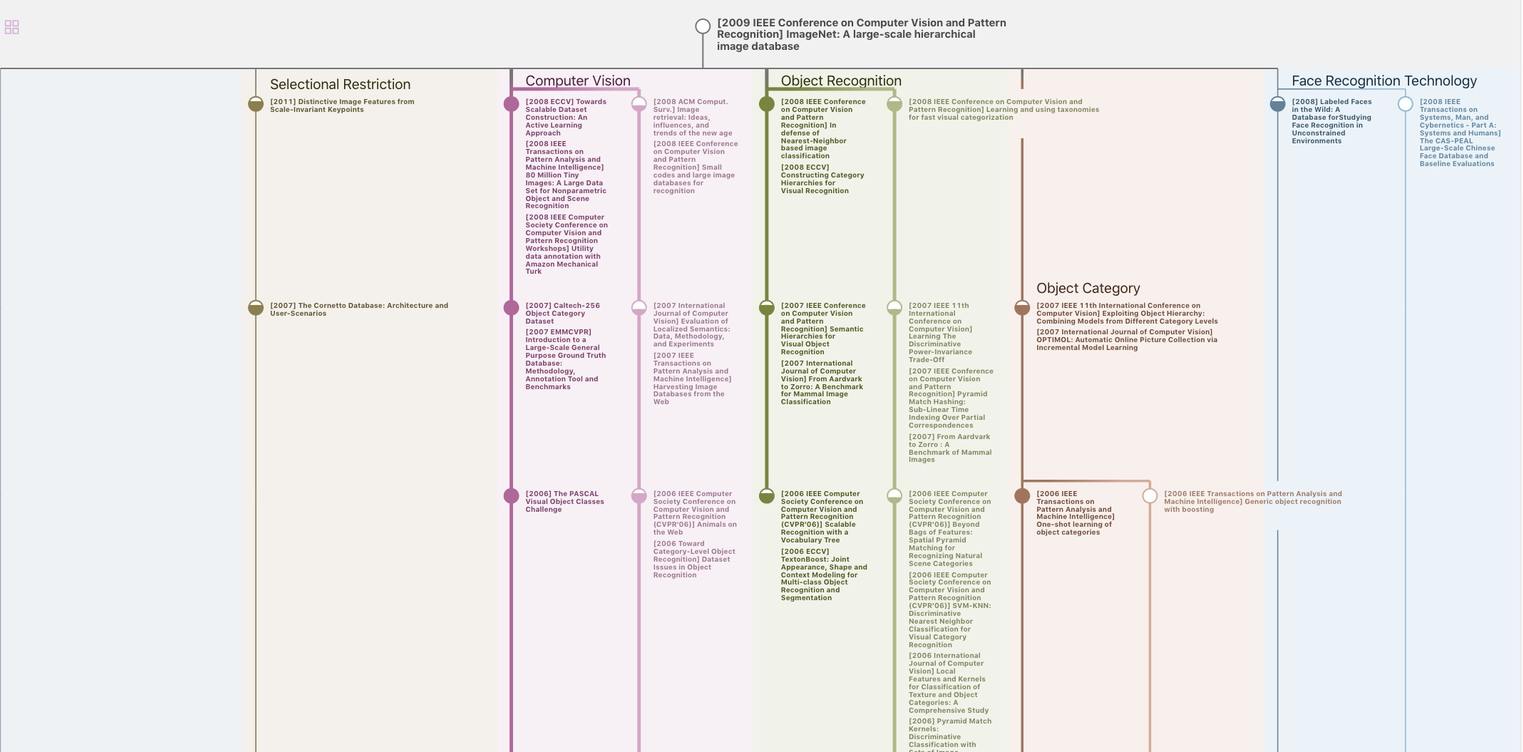
生成溯源树,研究论文发展脉络
Chat Paper
正在生成论文摘要