Mechanical induction of the tumorigenic [bgr]-catenin pathway by tumour growth pressure
Nature(2015)
摘要
To test the tumorous impact of early tumour growth pressure on non-tumorous epithelial tissues in vivo, apart from the mechanical stiffness characteristic of the microenvironment of late tumours1, 2, 3, 4, 5, 6 (see Supplementary Information 1), we first measured the strain deformation of live tumorous colonic crypts ex vivo under spinning disk microscopy. We used Notch/Apc mice, characterized by hyper-proliferative Notch overexpressing crypts within the Apc heterozygous genetic background (Notch+Apc+/1638N)7. Colon crypt stress was deduced from their strain deformation induced by Notch+ hyper-proliferative crypts, elasticity measurements, and Hooke’s law. It was found to range from 0 to 2.4 kPa at 2 weeks (S = 1.2 ± 1.2 kPa (mean ± s.d.)), and from 0.4 to 5.6 kPa at 1 month (S = 3 ± 2.6 kPa) (Supplementary Information 2 and Extended Data Figure 1a–c). These values are consistent with the pathophysiological oncogene growth-induced stress of a few kPa induced by tumour growth2, 8, 9. To apply and mimic a mechanical pressure in the same order of magnitude as the one generated by hyper-proliferative crypts in vivo, we developed a method based on the intravenous injection of stable ultra-magnetic liposomes (UML) encapsulating super-paramagnetic iron oxide nanocrystals. A 3-mm cylindric magnet was inserted subcutaneously in front of the colon, which caused loading of the colon tissue with the liposomes (Extended Data Figs 1d, 2a and Supplementary Information 3). Confocal microscopy showed UML loading, in the stromal cells surrounding the distal colon, one week after injection (Extended Data Fig. 2b). This remained stable, concentrated at 250 ± 50 nmol of Fe(III) per g of tissue, during 1 month (Extended Data Fig. 2c). Immuofluorescence tissue analyses revealed UML internalisation within the mesenchymal cells surrounding the crypts, as determined by vimentin co-labelling (Fig. 1a). The next step was to measure the tissue stress associated with the presence of UML exposed to a magnetic field gradient in the colon of wild-type mice. The compression strain generated by the 3 mm (0.12 T) magnet on the UML-loaded tissue along the y direction of the wild-type colon was found to be ε = 4.3 ± 2.1% (Fig. 1b, Extended Data Fig. 2a, d–f and Supplementary Information 4). Quantitative Young’s modulus maps of wild-type colons revealed a mean stiffness value of E = 30.1 ± 3.5 kPa (mean Young’s modulus), which was not significantly changed by magnetic loading to EUML = 35 ± 3 kPa (Extended Data Fig. 2g). These measurements enabled an estimation of a mean local uniaxial stress S = E × ε = 1.194 ± 0.610 kPa generated by the magnet on the colon, making the assumption of an almost uniaxial strain (along y direction from the colon to the magnet) and consequently a one-dimensional Hooke’s law (S = E × ε) approximation. This showed the existence of 100-µm acoustic resolution domains of stress ranging from 0.6 to 1.8 kPa. Notably, these values are consistent with the pathophysiological stress induced by tumour growth, as reported in the literature2, 8, 9. They are equivalent to the mechanical stress we measured in aberrant crypts generated in Notch/Apc mutant mice after 2 weeks to 1 month after aberrant crypt foci (ACF) development (Extended Data Fig. 1a). We then applied this magnetically induced pressure in vivo, to the colon of Apc single-mutant mice (Apc+/1638N), in which the β-catenin signalling pathway was specifically found to be mechanically activated in response to 20-min perturbation ex vivo10. This resulted in an oncogenic response leading to the increased expression of the β-catenin target tumorigenic Myc, Axin2 and Zeb110, 11, 12 after 1 month of pressure application in the crypts (Fig. 2 and Extended Data Fig. 3a). Quantification by qRT–PCR (quantitative reverse-transcriptase PCR) revealed an increase in expression of Myc, Axin2 and Zeb1 by factors of 2.3, 2.5 and 3.5, respectively, due to magnetic pressure mimicking growth-induced mechanical tissue stress (Extended Data Fig. 3a). Consistent with this transcriptional response, after 2 weeks of mechanical stress, we observed an increase of the phosphorylation of β-catenin on Y654 which prevents its interaction with junctional E-cadherin and allows its release from junctions13 (Fig. 2, yellow arrows). Consistently, the phospho-β-catenin did not co-localize with sub-apical junctional E-cadherins, but was detected more apically at the brush border, as assessed by co-localization with villin (Extended Data Fig. 4b). Apc-mutant crypts displayed an increased number of cells with a cytoplasmic enrichment in β-catenin, as well as nuclear enrichment, as shown by DNA co-staining with DAPI (Extended Data Fig. 3b, stained in white), attesting β-catenin accumulation both in the cytoplasm and nucleus in intestinal epithelial cells14. Consistent with the mechanical induction of Myc (Fig. 2), we observed an increase in the number of Ki67-positive proliferative cells (by 1.5-fold compared to control mice) after 2 weeks of mechanical stress (Extended Data Fig. 4a and Supplementary Note), and a consequent increase in the number of crypts larger than 1,500 μm2 (Extended Data Fig. 3c). This corresponded to an increase in mean crypt size of 22% after 1 month, which is similar to the value recorded during the appearance of ACF in Notch/Apc mice, 1 month after Notch activation (Extended Data Fig. 3c). Using small-animal colonoscopy, we could visualize the mechanical induction of ACF initiating 5 days after stress application, leading to adenoma development after 15 days in Apc+/1638N mice (Extended Data Fig. 5a, b and Supplementary Information 5 with associated Extended Data Fig. 2c, h, i). Notably, no loss of Apc heterozygosity15 could be detected in the epithelia in response to mechanical pressure at this time, in agreement with mechanically induced ACF formation (Extended Data Fig. 5c). Therefore, a magnetic pressure mimicking the strain generated by tumour growth triggered the activation of the Wnt/β-catenin pathway, leading to the mechanical induction of Myc, Axin2 and Zeb1 expression, genes critically involved in tumour growth, and to ACF formation in Apc+/1638N mice in vivo. Then, we aimed to gain insights into the molecular mechanisms underlying the observed pro-tumorigenic effects of magnetically induced pressure. We found Ret mechanical phosphorylation as required upstream of the tumorigenic mechanosensitive pathway, ex vivo (Extended Data Figs 4d, e, g, 6a, b and Supplementary Information 6). To test whether tumour growth pressure could activate Ret in vivo, we first applied the magnetically induced pressure mimicking the σ = 1.194 ± 0.660 kPa tumour-growth-induced stress in vivo, to heterozygous Apc+/1638N mutant mice. We observed Ret phosphorylation in Apc+/1638N crypts at 1 week of induced mechanical stress (Fig. 3 and Extended Data Fig. 6c), initiating as early as after 30 min coincidental with UML accumulation (Extended Data Fig. 7a, b and Supplementary Information 6), consistent with the rapid dynamics associated with mechanotransductive processes and a direct mechanical activation of Ret. Ret activation, and downstream β-catenin Y654 phosphorylation and Myc induction, were all found to be independent of any indirect effect of UML loading per se (Figs. 2, 3 and Extended Data Fig. 7c, d), or long-range effects of liposomes or magnets either in the colon or outside the colon (Supplementary Information 7). We consistently found no expression of the Ret canonical ligands in the colon GDNF, artemin and neurturin (Extended Data Fig. 7e), which confirmed Ret mechanical activation in these experimental conditions. We additionally found Akt-dependent Ret mechanical activation upstream of GSK-3β inactivation, a protein that prevents nuclear accumulation of the β-catenin released into the cytoplasm (Extended Data Fig.7f, g and Supplementary Information 8)16, 17. Importantly, when we applied a magnetic pressure generating a tissue stress corresponding to S = 1.194 ± 0.660 kPa to wild-type colon, we obtained the same results as in Apc+/1638N mice, namely Ret activation, β-catenin phosphorylation and release from sub-apical junctions and co-localized with villin and increased nuclear translocation of β-catenin (Extended Data Figs 4c and 6d, e). The observation of Myc overexpression and ACF formation with induced internal growth pressure were also observed, at 2 and 3 months, respectively (Extended Data Figs 6d, e, f and Supplementary Information 9), with no loss of the Apc allele in wild-type mice (Supplementary Information 9). Finally, we tested whether such a mechanotransduction pathway was activated by the endogenous physical pressure induced by hyperproliferative effects of Notch-activated crypts on Notch-negative crypts, in Notch/Apc mutant mice colon. First, we found that both Ret and β-catenin were widely phosphorylated in aberrant Notch/Apc mutant colonic crypts. Enrichment in cytoplasmic and nuclear β-catenin were also observed 1 month upon Notch activation as well as Myc overexpression after 2.5 months (Extended Data Figs 8a, b, 4f and Supplementary Information 10). Specifically, Ret phosphorylation, β-catenin phosphorylation and nuclear translocation, as well as Myc overexpression were observed not only in Notch-expressing crypts, but also frequently in non-tumorous crypts lacking Notch activation (Fig. 4 and Extended Data Fig. 8a, b). A substantial number of these crypts, which activates Ret, are surrounded by Notch negative crypts (Extended Data Fig. 9a). This is in agreement with long-range non cell-autonomous mechanical induction in healthy tissue by the strain developed by distant Notch hyperproliferative domains (Supplementary Information 11 with Extended Data Fig. 9a, b, c, d). No exonic mutations were detected in Ret in Notch/Apc mice epithelia or in UML + magnet Apc+/1638N mice, 3 months after tamoxifen injection and magnetic pressure, respectively, as well as in the wild-type copy of Apc+/1638N (not shown). Altogether, our results show the mechanical activation of the tumorigenic Ret/β-catenin pathway in response to the pressure characteristic of hyperproliferative tumour growth pressure in colon epithelia in both Apc+/1638N and wild-type mice. We found that such a mechanotransduction process also takes place in non-tumorous Apc+/1638N heterozygous crypt cells compressed by Notch+ hyperproliferative crypts of Notch/Apc mice (Supplementary Discussion 1). Based on these findings, we postulate that mechanical stimulation of tumorigenic pathways could potentially occur in normal tissues neighbouring any type of tumour, contributing to an unstable positive feedback loop between oncogene expression and tumour induction, enhancing the growth and spread of the tumour (Supplementary Discussions 1, 2, 3 and Extended Data Fig. 10 a–c). Detailed protocols are deposited in Protocol Exchange, http://dx.doi.org/10.1038/protex.2014.052. All mice used in this work have been previously described: Apc+/1638N (ref. 18), VilCreERT2/Nic and VilCreERT2/Nic;Apc+/1638N (ref. 7), including males and females. C57Bl/6 inbred mice were obtained from Charles River Laboratories and females were used as wild-type controls. For the experiments on VilCreERT2/Nic;Apc+/1638N mice, four-week-old mice were injected intraperitoneally with tamoxifen (ICN) (50 µg g−1 of animal body weight) for five consecutive days. All animal experiments conformed to NIH guidelines for the care and use of animals and were approved by the local ethics committee (Approval no. 2012-0016). In this mouse model, a tamoxifen-inducible Cre recombinase (CreERT2) expressed under the control of the intestinal-specific villin promoter induces the expression of an intracellular and constitutively active form of the mitotic Notch 1 receptor (N1IC), along with a nuclear GFP reporter. As the VilCreERT2 mice are mosaic, tamoxifen injection results in clones of mitotic cells expressing N1IC (N marked by GFP) within Apc+/1638N heterozygous colonic cells. The aqueous suspension of magnetic nanoparticles was prepared using alkaline co-precipitation of FeCl2 (0.9 mol) and FeCl3 (1.5 mol) salts, according to Massart’s procedure19. Superparamagnetic maghemite grains (γ-Fe2O3) were obtained by oxidizing 1.3 mol of magnetite with 1.3 mol of iron nitrate (boiling solution). After magnetic decantation, 2 l of distilled water and 360 ml of HNO3 20% were added to the solution and the mixture was stirred for 10 min. Prepared maghemite nanoparticles were washed several times with acetone (3 × 1 l) and ether (2 × 500 ml) and suspended in water. Size sorting was performed by adding HNO3 (0.45 M) to the suspension followed by magnetic decantation. This operation was repeated with the deposit until a suitable particle size was obtained. Sodium citrate (nFe/nCit = 0.13, molar ratio) was added to the nanoparticles and the mixture was heated at 80 °C for 30 min to promote absorption of citrate anions onto their surface. Citrated nanoparticles were precipitated in acetone and suspended in water. The volume fraction and average size of the maghemite grains were determined by fitting the magnetization curve of nanoparticles using Langevin’s Law. Particles of 7.7 nm (standard deviation σ = 0.37) and of 9 nm diameter (standard deviation σ = 0.35, volume fraction of nanoparticles in the suspension ϕ = 1.9%, specific susceptibility χ/ϕ of 15.5) were obtained and used for magnetic liposomes and ultra-magnetic liposomes (UMLs), respectively. The aqueous medium was removed using a Macrosep advance centrifugal device (PALL) and nanoparticles were suspended again in a buffer (0.108 M NaCl, 0.02 M sodium citrate and 0.01 M HEPES, pH = 7.4). Solutions of 1,2-dipalmitoyl-sn-glycero-3-phosphocholine (DPPC), 1,2-distearoyl-sn-glycero-3-phosphocholine (DSPC), 1,2-distearoyl-sn-glycero-3-phosphoethanolamine-n-[(carboxy(polyethyleneglycol)2000](ammonium salt) (DSPE-PEG2000) and l-α-phosphatidylethanolamine-N-(lissamine rhodamine sulfonyl B) (ammonium salt) (rhodamine-PE) in chloroform were purchased from Avanti Polar Lipids, Inc. UML were prepared by the reverse phase evaporation method established in ref. 20 and modified according to a previously described protocol21. In brief, a mixture of DPPC/DSPC/Rhod-PE/DSPE-PEG2000 (84/10/1/5 mol%, 315 μl) was dissolved in 3 ml of diethyl ether (VWR) and 900 µl of chloroform (Carlo Erba reagents). Thereafter, 1 ml of citrated magnetic nanoparticles dispersed in the buffer was introduced before sonication at room temperature for 20 min to produce a water-in-oil emulsion. Organic solvent was evaporated with a rotavapour R-210 (Buchi) at 25 °C until the gel phase disappeared. Liposomes were filtrated through a 450 nm filter and purified from non-encapsulated magnetic nanoparticles by magnetic sorting using a strong magnet (Calamit Fe−Nd−B 150 × 100 × 25 mm). The operation was repeated three times every 2 h and liposomes (highly concentrated at 30% of nanoparticles in volume) were finally separated from the supernatant and recovered. Magnetic liposomes were prepared according to a procedure already described22, 23, 24, 25, 26. In brief, the magnetic fluid used to load the liposomes consisted in an aqueous suspension of 8-nm nanocrystals of maghemite (γ-Fe2O3) synthesized according to the procedure described above. In these conditions, superparamagnetic grains (from magnetization curve22, 23) were produced. Final adjustment of both aqueous medium and maghemite concentration (5.4 ± 0.1 M Fe(III), checked by flame spectrometry) was performed by ultrafiltration (MACROSEP filter, cut-off 50 kD, Fisher Scientific Labosi) followed by addition of the same buffer as that used for liposome preparation (0.108 M NaCl, 0.02 M sodium citrate and 0.01 M HEPES, pH = 7.4). Final magnetic fluid was composed of nanoparticles of 20.3 nm in hydrodynamic diameter (0.1 polydispersity index from QELS measurements) and stabilized by adsorption of citrate anions onto their surface. Rhodamine-labelled magnetic-fluid-loaded liposomes (MFLs) were prepared by hydration of a thin lipid film (EPC:DSPE-PEG2000:rhodamine-PE; 94:5:1 mol%, determined by weight, precision of 5.10−5 g) with equal volumes of magnetic fluid and buffer to get a total lipid concentration of 20 mM (checked by fluorescence spectroscopy). Hydration was followed by sequential extrusion under nitrogen pressure (<10 bars) at 25°C through polycarbonate filters with decreasing pore diameters of 0.8 μm/0.4 μm/0.2 μm (PORETICS, Osmotics, Livermore, USA). Liposomes of 226 ± 38 nm in hydrodynamic diameter (from QELS measurements) were recovered. Non-entrapped maghemite particles were separated by gel exclusion chromatography (GEC) performed with a 0.4 cm × 5.8 cm Sephacryl S1000 superfine (Pharmacia) microcolumn (TERUMO 1 mL-syringe) beforehand saturated with EPC: DSPE-PEG2000 (95:5 mol%) liposomes, prepared similarly to MFLs but without maghemite. The eluent was the buffer used for liposome preparation. Final Iron content of the preparation was checked by ESR analysis and found equal to 44 ± 1 mM [Fe(III)], that is, 2.2 iron to lipid molar ratio (0.7% in volume concentration of nanoparticles). Hydrodynamic diameters were determined by using a photon correlator spectrometer (10 mW HeNe 632.8-nm laser, Zetasizer Nano ZS90, Malvern Instruments) at T = 25 °C and 90° scattering angle from the measured translational diffusion coefficients of the particles according to the Stokes–Einstein law for non-interacting spherical particles. Just before measurements, the samples were diluted with buffer to optimize the response of the apparatus. Unimodal distribution analysis was used and measurements were performed in triplicate. UMLs and MLs were injected intravenously in Apc+/1638N and wild-type mice at 3–4 months (Extended Data Fig. 2a). The essential prerequisite of the experiments was to develop a methodology for introducing magnetic particles into the mesenchymal tissue of the colon at the intracellular level and at concentrations sufficiently high to allow subsequent magnetic manipulation. Furthermore, the mode of delivery of the magnetic material must preserve the tissue from any other stress, which would become competitive with the mechanic constraint generated magnetically. Thus the delivery must be realized through an indirect route of administration. Systemic delivery via intravenous injection performed outside the region of the colon ranks among the best ways provided that the pharmacokinetics of the magnetic particles is optimized to reduce first-pass hepatic clearance and permit observable distribution in the colon tissue. In this respect, we used submicron liposomes sterically stabilized by polyethylene glycol (PEG) coating as bioavailibity-enhancing carrier of the magnetic particles. Indeed, PEGylated magnetic-fluid-loaded liposomes not exceeding 200 nm in diameter have reliably been proved to be long-circulating systems as intact vesicle structures without leakage of their inner content, therefore aptly averting dilution of the magnetic material22, 23, 24. Moreover they have shown to diffuse from the vasculature into the interstitial tissues without loss of structure integrity and at the intracellular level as well25, 26. Said otherwise, the containment of the magnetic particles required for magnetic manipulation and beforehand adjusted within the liposomes is totally conserved during their passage through the vascular endothelium towards the surrounding tissues and upon cellular uptake. Ex vivo global compression. To apply an ex vivo mechanical compression colon samples were treated as previously described with a confinement box of 3 mm thickness for 0.8 kPa pressure applied10. For kinase inhibition experiments, PP1, PP2 (30 μM; BioSource), SU6656 (20 μM; Sigma-Aldrich), sunitinib (20 μM; Sigma-Aldrich), vandetanib (10 μM; Selleck Chemicals), ponatinib (100 nM; Selleck Chemicals), or an equivalent volume of the vehicle DMSO (diluted to 1 in 500), was added to reduced serum medium 20 min prior starting the compression. In vivo magnetic deformation. Mice were anaesthetized with isofluorane (delivered at 2% for maintenance and 1.5% for induction in oxygen) and a 3 mm diameter strong magnetic field gradient magnet positioned subcutaneously on the back of the mice in front of the colon (Extended Data Fig. 2a). The fluorescent UML (associated with rhodamine-PE phospholipids) were diluted in a 10 mM HEPES pH 7.4, 20 mM Na3Citrate, 108 mM NaCl buffer solution to a final concentration of 0.1 M and injected in the lateral caudal veins of the tail. The only rare side-effect of injection was a small hematoma that disappeared in two to three days later. Magnet implantation caused only local skin inflammation that was treated with antiseptics and disappeared in three to four days. No effect on intestinal transit was detected nor dysfunction of the colon, which was largely isolated from skin. The Src family inhibitors used in this study are PP1 and PP227, and SU6656 as a specific inhibitor of Src28. The inhibitors of Ret used in this study are sunitinib29, vandetanib30 and ponatinib31, 32. The distal colon was dissected from adult mice, rinsed with PBS and incubated at 37 °C in Leibovitz’s L-15 medium + Glutamax-1 supplemented with 10% fetal bovine serum and 40 μg ml−1 gentamicin. Individual tissue segments were opened longitudinally and placed villi up between two cover-slides. Images were obtained with an inverted Olympus IX70-Ropper spinning disc microscope coupled to a Cool Snap HQ2 camera. Iron concentration analyses were realized by electron spin resonance (ESR) analysis. UML and magnetic liposome biodistribution was assessed by determining the amount of iron oxide in excised tissues using ESR spectrometry. ESR spectra were acquired using an Elexsys E500 ESR spectrometer (Bruker Biospin) operated at: resonant frequency ≈ 9.2 GHz; microwave power = 10.11 mW, receiver gain = 60 db and magnetic field modulation amplitude = 10G, at room temperature. After the sacrifice of the mouse, distal colon samples were lyophilized for 24 h. Maghemite nanocrystal suspensions used for each liposome sample and of known iron concentration were used as standards to construct a calibration curve (R2 = 0.99) for quantitative purposes. ESR spectra were collected as the first derivative of the absorbed microwave power versus the magnetic field. A double integration was applied to obtain the area under the curve of the absorption-field curve, which is proportional to the number of resonating electronic spins in the measured sample. All tissue samples studied were corrected for background tissue absorption of the microwave radiation using tissue samples of control mice not exposed to UML. All the double integrations were calculated over the field interval (1500–5500 Gauss). Iron amounts were normalized by tissue sample weights (mol of Fe per mg tissue). Iron concentration in Apc+/1638N at 1 week was confirmed to be on the same order of magnitude than at 1 week in the wild type before being quantitatively evaluated for each time point conditions based on the quantitative comparison of the mean density of fluorescence associated to UML between each Apc+/1638N sample, and the mean fluorescence value of the wild-type samples (using ImageJ), based on ten immunohistological samples per mouse. A distal colon explant was completely embedded in an agar–gelatin phantom. The ultrasonic probe was fixed on one side of the phantom and a small magnet approached axially towards the colon by a support. To measure the axial deformation (along y direction), strain imaging is performed, by acquiring an ultrasonic image before and after the magnet was displaced. The magnetic-force-induced tissue strain, which was measured by estimating local tissue displacement along the y direction from the first acquisition to the second one. Note that the time between moving the magnet and acquiring the data is less than 5 s, which is short enough to avoid creep behaviour. Average values were obtained from at least four measurements for each condition. Shear wave elastography (SWE) was performed to image quantitative tissue stiffness. A remote and tiny palpation (tens of µm) is induced by the acoustic radiation force of an initial focused ultrasonic beam and this radiation force generates a shear wave propagating along the x direction. Tissue displacements induced by this shear wave are mapped by performing ultrafast ultrasonic imaging. The local shear wave speed is linked to stiffness. Dissected colon samples were embedded in agar–gelatin phantoms (2% agar with 5% gelatin)33. Ultrasound images, so called B-mode and elasticity images were acquired using a high frequency ultrasound probe (15 MHz, 256 elements, Vermon) driven by an ultrafast imaging device (Aixplorer, Supersonic Imagine)34. SWE, that is, Young’s modulus (E) quantitative imaging, was performed by using the supersonic shear wave imaging (SSI) technique ex vivo and in vivo35, 36. The SSI technique is based on the ultrafast ultrasound imaging of a shear wave induced by the radiation force of an initial focused ultrasonic beam acting as a remote palpation in tissues (Extended Data Fig. 2e). Under the assumptions of a local constant density ρ and a locally incompressible and isotropic elastic medium, the propagation speed vs of the tracked shear wave is directly linked to the Young’s modulus E (in kPa) characterizing the local stiffness via the relationship: E = 3ρ × vs2. The assumption of constant density of in vivo soft biological tissues here is valid. Indeed, in biological soft tissues, the density is almost constant ρ ≈ 1,000 kg m−3 due to the very high water content of soft tissues. However, small density variations exist as shown in extensive past studies37, 38. From these studies, the mean density (among connective tissues, muscle, fat, blood cells, plasma, cornea, spinal cord, spleen, testis) is 1,052 ± 47 kg m−3. Thus, the normalized standard deviation in soft tissues is 4.7%. Also, due to their high fluid content, many soft biological tissues and gels exhibit nearly incompressible behaviour under physiological loading; they are constrained to undergo essentially volume-preserving deformations and motions. Thus, it is commonly accepted in the field of tissue elasticity measurements that the Poisson’s ratio of tissue has a value between 0.49 and 0.4999, meaning that tissue is nearly incompressible39, 40. Of course, this incompressible behaviour is only ensured provided that the conditions of interest do not allow the water to diffuse into or out of the tissue during the period of interest. This is the case in the SWE approach, due to the very small (micrometric) displacements induced by the shear wave used to probe local elasticity. Such tiny displacements do not induce water diffusion outside of the organ. Although the assumption of local isotropy has to be done to derive the Young’s modulus from the shear wave speed measurements, it is not possible currently to prove its validity in vivo. In particular tissue such as muscles, an elastic anisotropy has already been proven in vivo41, 42. However, the in vivo assessment of such anisotropic elasticity was only made possible in particular configurations, such as the human biceps, because the structural organization and orientation of the muscular fibre bundles is highly identical over a large region of interest. Other tissues like breast, arteries, liver are today assumed as isotropic in the field of elastography. Even if a local anisotropy could potentially exist in these tissues, we postulate that it should remain quite small. Indeed, it was recently shown that the anisotropy of shear modulus in the in vivo kidney was quite small with a fractional anisotropy of cortex and medulla <20% (see Fig. 3. of ref. 43) despite a more important tissue organization in the kidney (due to the alignment of the pyramids) than in the other tissues. Extensive calibration experiments were performed in the past to demonstrate the ability of SWE to quantify the Young’s modulus of tissues. The standard deviation of Young’s modulus quantification was demonstrated to be lower than 5% on calibrated phantoms mimicking biological tissue properties44, 45. A small magnet (3 mm in diameter) was axially approached towards the colon by steps of 0.5 mm until completing 3 mm of absolute axial displacement. For each position of the magnet, strain images (ε) were calculated by comparing raw frequency ultrasound images acquired at two consecutive steps46. Cumulative one-dimensional strain along the y direction was obtained by summing all strain images (Extended Data Fig. 2f). Although the magnet could induce some stress in the full volume in the three directions of space, it is here considered that the strain in the lateral (x) and elevational (z) directions remain small compared to the measured axial (y) strain. Under this assumption of a force that is in majority in the axial y direction, the quantitative stress S applied by the magnetic field acting on ferrofluids trapped in colon tissues was retrieved by calculating the one-dimensional Hooke’s law: S = E × ε (ref. 47). Using Hooke’s law, the standard deviation of S was experimentally estimated and found to be equal to 0.61 kPa. It is in good agreement with a 0.74 kPa standard deviation of S derived from equation (1) that describes the influence of E and ε uncertainties (35.0 ± 3.0 kPa and 4.3 ± 2.1% ,respectively) on the uncertainty of S: During the experiments, collection was performed no more than 4 s after the compression induced by the magnet motion. Such a small delay ensures that one can avoid any creep behaviour. Indeed, the relaxation time for typical human tissues under compression is of the order of several tens to hundreds of seconds48. The distance between the colon and the magnet was evaluated using ultrasound imaging in vivo, with anal injection of a water gel transparent to ultrasound in the colon lumen to visualize the colon. Isolation of epithelial cells of the colon was performed by incubation of tissue explants in 3 mM EDTA and 0.5 mM DTT in phosphate-buffered saline (PBS) for 45 min. Cell pellets were washed in PBS and lysed in radioimmune precipitation assay buffer (50 mM Tris-Cl (pH 8.0), 150 mM NaCl, 1% Nonidet P-40, 0.5% deoxycholate and 0.05% SDS) plus 1% phosphatase inhibitor cocktail, and 1% protease inhibitors. The protein content of the supernatant was measured by the colorimetric reaction RD DC protein assay (Bio-Rad). An equivalent quantity of protein (20 µg) was resolved by SDS–PAGE, transferred to a nitrocellulose membrane (Invitrogen), and hybridized with the appropriate antibodies, followed by detection using enhanced chemiluminescence (Pierce ECL Plus Western Blotting Substrate, Thermo Scientific). Protein levels were quantified using ImageJ. The following antibodies were used: anti-pY1062 Ret (1:100, Santa Cruz Biotechnology), anti-GAPDH (1:5,000, abcam), and horseradish peroxidase (HRP)-conjugated secondary antibodies (1:1,000, Jackson ImmunoResearch). Total RNA isolation was performed by using NucleoSpin RNAII (Macherey-Nagel), according to the manufacturer’s instructions and quantified with a NanoDrop ND-1000 spectrophotometer. First-strand cDNA was synthesized by using AccuScript High Fidelity 1st Strand cDNA Synthesis kit (Agilent Technologies). For qPCR, reactions were run on a real-time PCR system (ABI Prism 7900; Applied Biosystems) and gene expression was detected with Power SYBR Green (Applied Biosystems). Relative gene expression was determined by normalizing to reference genes β2-microglobulin and TATA box binding protein TFIID (transcription factor IID), using the comparative threshold cycle (CT) method49. Results were expressed as fold change of each sample versus control. The primers used in each reaction were as follows: Myc forward 5′-TCAAGAGGCGAACACACAAC-3′ and reverse 5′-GGCCTTTTCATTGTTTTCCA-3′; Axin2 forward 5′-CGCCACCAAGACCTACATACG-3′ and reverse 5′-ACATGACCGAGCCGATCTGT-3′; Zeb1 forward 5′- TGGCAAGACAACGTGAAAGA-3′ and reverse 5′-AACTGGGAAAATGCATCTGG-3′; TFII forward 5′-CCACGGACAACTGCGT-3′ and reverse 5′-GGCTCATAGCTACTGA-3′; β2-microglobulin forward 5′-GCTATCCAGAAAACCCCTCAA-3′ and reverse 5′-AGGCGGGTGGAACTGTGTT-3′. Statistical data analyses were performed using a two-tailed unpaired Student’s t-test between any two groups (n = 7). The UniProt accession numbers for sequences used in the gene expression analyses are: Axin2, O88566; Myc, P01108; and Zeb1, Q64318. Targeted re-sequencing of Apc and Ret gene panel was designed with Ion ampliseq designer v3.2. Multiplex PCR primers were selected to amplify the coding sequences of the selected genes: Apc (98,39% of the gene coding sequence covered by the design), Ctnnb1 (100%), Ret (99,8%), Gsk3b (100%) and Axin2 (96,26%) with an exon padding of 25 bp. Sequencing libraries were built with the Ion Ampliseq Library Kit v2.0 following manufacturer’s recommendations (Life Technologies). In brief, 10 ng of each DNA sample was mixed with designed primer pools and supplied PCR mix to amplify targeted genomic regions. PCR products corresponding to PCR primers were partially digested and barcoded Ion adapters were ligated at the 5′ and 3′ extremities of digested PCR products. After purification of ligation products, PCR amplification followed by quality control was performed. Generated sequencing libraries were then pooled in equimolar ratio and sequencing templates were prepared on an Ion One Touch system with the Ion OneTouch 200 Template Kit v2 DL (Life Technologies). After selective enrichment and loading on a Ion 318v2 chip, positive sequencing templates were sequenced on a Ion Torrent PGM with the Ion PGM Sequencing Kit 200 v2 (Life Technologies). Three technical replicates for each mouse DNA sample were processed in parallel to determine the technical variability to accurately evaluate the copy number variation on the targeted genes. Sequenced reads were aligned on the mm10 reference genome using the TMAP software (v3.6.2, Life Technologies). Around 95% of the reads were aligned on the targeted genes with a mean depth of coverage of 1750×. The variant calling was performed using the Ion Torrent Variant Caller (v3.6.2, Life Technologies) and annotated with the RefSeq genes and dbsnp138 databases using the Annovar software50. Variants found with a low coverage (≤30×) or with a strand bias were filtered out. Isolation of epithelial cells of the colon was performed by incubation of tissue explants in 3 mM EDTA and 0.5 mM DTT in PBS for 45 min. The supernatant was then discarded, 10ml of cold PBS added, and the mixture was shaken vigorously by hand before being spun for 20 min at 153g at 4 °C to obtain the cell pellet. Total DNA isolation was performed by using QIAamp DNA mini kit (Qiagen), according to the manufacturer’s instructions. For PCR, gene expression was detected with BioTaq Red DNA Polymerase (Abcys). For Apc genotyping, the following primers were used: primer PA2, TCAGCCATGCCAACAAAGTCA, primer PN3, GCCAGCTCATTCCTCCACTC and primer C2, GGAAAAGTTTATAGGT. Cycling conditions were 5 min at 94 °C (1 cycle); 30 s at 94 °C, 45 s at 59 °C, and 345 s at 72 °C (35 cycles); and 5 min at 72 °C (1 cycle). The presence of the wild-type allele is indicated by a 300-bp PCR fragment (primers PA2 and C2) and the mutant allele by a 400-bp PCR fragment (primers PA2 and PN3). Isolation of epithelial cells of the colon was performed by incubation of tissue explants in 3mM EDTA and 0.5mM DTT in phosphate-buffered saline (PBS) for 45 min. The supernatant was then discarded, 10ml of cold PBS added, and the mixture was shaken vigorously by hand before being spun for 20 min at 153g. at 4 °C to obtain the cell pellet. DNA was isolated according to the QIAamp DNA mini kit (Qiagen) in order to be analysed by the Sequencing Platform of the Institut Curie. A dedicated sequencing panel targeting the coding sequence of the Apc and Ret genes was used. Only one variant (D2086G) was detected in the Apc gene in one control mouse and one mouse subject to mechanical pressure. This single nucleotide change is referenced as a common polymorphism in the dbSNP database (rs47505115). No Y1062 mutation was detected in the Ret gene. No exonic mutation was detected in the Ret gene (not shown). Freshly dissected colon samples were treated as previously described10. The commercial antibodies used were: anti-Myc N262 (1:50, Santa Cruz Biotechnology), Twist H81 (1:50, Santa Cruz Biotechnology), β-catenin (1:100, BD), pY654 β-catenin (1:50, abcam), pY1062 Ret (1:50, Santa Cruz Biotechnology), Zeb1 (1:50, Santa Cruz Biotechnology), vimentin (1:300, Sigma), Ki67 (1:200, abcam), pS9 GSK-3 (1:50, Cell Signalling), pY568/570 Kit (1:100, Santa Cruz Biotechnology), pY412 Abl (1:50, Santa Cruz Biotechnology), pY209/211 Hck (1:50, Sigma), pY537 Yes (1:100, Santa Cruz Biotechnology), pY1238/1239 Ron (1:50, Santa Cruz Biotechnology), pSer473 Akt (1:50, Santa Cruz Biotechnology), E-cadherin (1:100, Santa Cruz Biotechnology), villin (1:100, Santa Cruz Biotechnology), GDNF (1:50, abcam), neurturin (1:200, abcam), artemin (1:200, abcam), anti-mouse Cy3 (1:500, Jackson ImmunoResearch), anti-rabbit Alexa 568 (1:600, Molecular Probes), anti-rabbit Alexa 350 (1:200, Molecular Probes) and anti-rabbit Alexa 488 (1:200, Molecular Probes). All images were taken with a Zeiss LSM710 microscope at the Platform for Cell and Tissue Imaging of the Institut Curie. Nuclear β-cat translocation was evidenced using the co-localization highlighter function of the co-localization analysis ImageJ plugin (http://www.uhnresearch.ca/facilities/wcif/imagej/colour_analysis.htm). Co-localization was coded in a binary way as white pixels (positive for co-localization) and quantified by measuring the density of white pixels, with control parameters adjusted at the threshold showing no co-localization in controls. All quantitative results were analysed using the non-parametric Mann–Whitney exact test and Student’s t-test, both two-tailed, with standard deviation error bars being comparable within each group of data. All experiments were reproduced at least twice in the laboratory. n is the number of measurements by experiment. No randomization was performed. No blinding was performed. No statistical method or power analysis was used to predetermine sample size. The coordination of the distinct disciplinary aspects of the work (acoustic, magnetism, magnetic and tamoxifen injections, surgery, labelling, qPCR, etc.) between the ten groups involved was complex, and did not materially allow us to organize such procedures. Download references This research was funded by the CNRS, INSERM, ARC (grant no.s 5030 and 29324), ANR (grant no.s 09PIRI0013-01 and 11 BSV5014-01), the Labex CelTisPhyBio (grant no. 11-LBX-0038), Fonds CSP, Fondation Pierre-Gille de Gennes, Fondation de France and the Inca (grant no. PLBIO13-172). High-throughput sequencing was supported by the grants ANR-10-EQPX-03 and ANR10-INBS-09-08 from the Agence Nationale de la Recherche (investissements d’avenir) and by the Canceropôle Ile-de-France. We thank the members of the Animal House Facility of Institut Curie, particularly Stéphanie Boissel, Virginie Dangles-Marie and Isabelle Grandjean, the IC Imaging facility members of PICT-IBiSA at BDD (UMR3215/U934) and Cell and Tissue Imaging Facility (UMR144 CNRS), Olivier Renaud’s team and François Waharte, Thibaut Brunet for discussions on GSK3 phosphorylation, Pierre Gestraud for NGS analysis help, Jean-Michel Frapart and Sonia Lajnev for help in the RPE experiments, and Didier Meseure (IC hospital) for anathomo-pathological analyses of the mechanically induced tumours.
更多查看译文
关键词
Cancer microenvironment
AI 理解论文
溯源树
样例
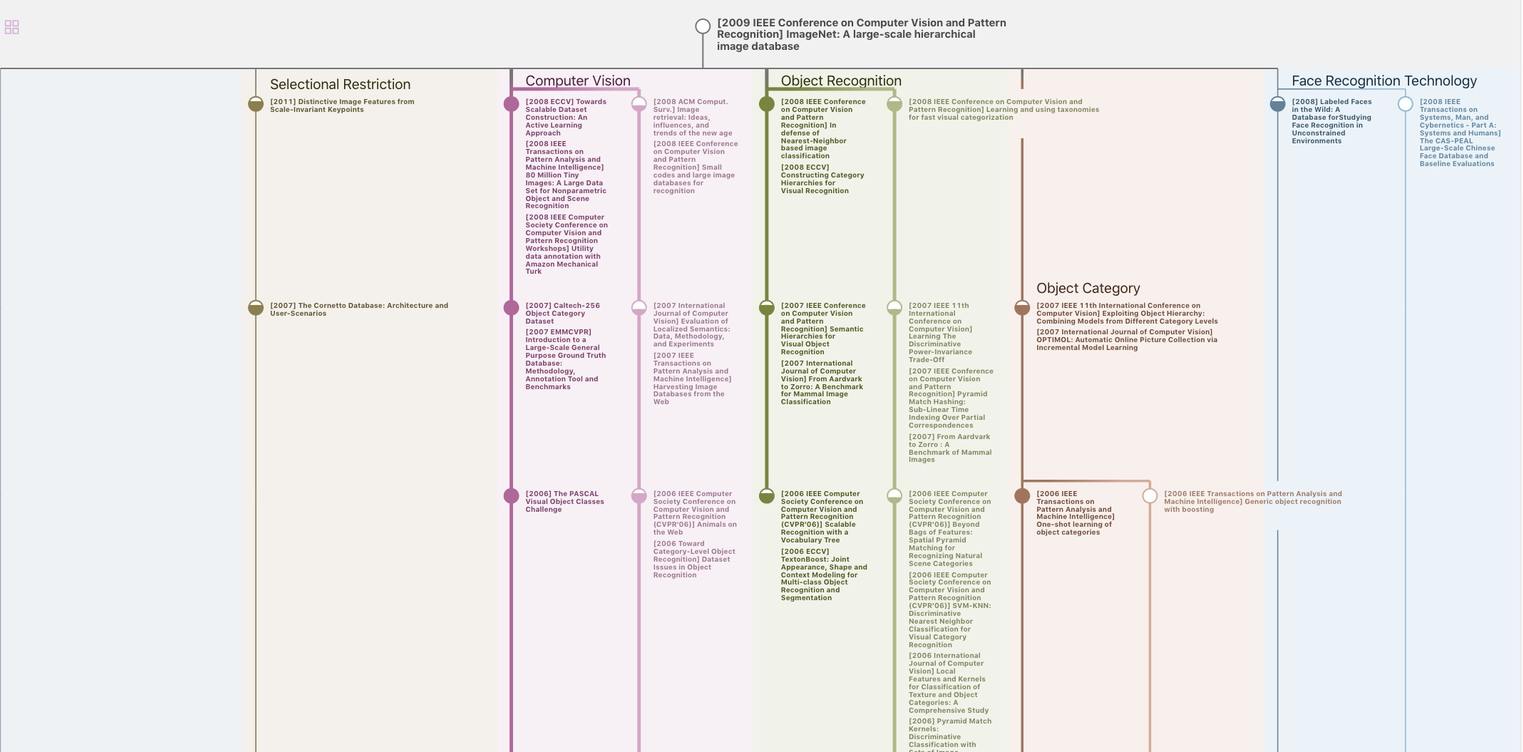
生成溯源树,研究论文发展脉络
Chat Paper
正在生成论文摘要