C.[thinsp]elegans Punctin specifies cholinergic versus GABAergic identity of postsynaptic domains
Nature(2014)
摘要
In C. elegans each body-wall muscle cell receives excitatory and inhibitory inputs from cholinergic and GABAergic motoneurons, respectively4. Acetylcholine (ACh) and GABA ionotropic receptors cluster in distinct subcellular domains opposing the corresponding neurotransmitter release sites. Although the molecular mechanisms for GABAA receptor (GABAAR) clustering at GABAergic NMJs are not known, genetic screens have identified three proteins expressed by muscle cells that are necessary for the synaptic clustering of heteromeric levamisole-sensitive acetylcholine receptors (L-AChR)8, 9, 10, 11. LEV-10 is a transmembrane protein with a large extracellular region containing five CUB (complement, urchin epidermal growth factor, bone morphogenetic protein) domains12. LEV-9 and OIG-4 are secreted proteins containing CCP (complement control protein) and immunoglobulin domains, respectively13, 14. These proteins form an extracellular scaffold in the synaptic cleft that physically interacts with L-AChRs. Neither L-AChR, LEV-9 nor LEV-10 is localized at the synapse by itself, suggesting that additional determinants specify the synaptic localization of this molecular complex. To identify the synaptic organizers controlling the formation of excitatory synapses, we performed a visual screen for abnormal L-AChR distribution with the use of a knock-in strain in which the red fluorescent protein TagRFP was introduced into the genomic locus of the essential L-AChR subunit unc-29 (refs 15, 16). In this strain, UNC-29–RFP accurately localizes at cholinergic NMJs, and synaptic L-AChR clusters can be observed in vivo by epifluorescence (Fig. 1a). We isolated a mutant allele of the Ce-Punctin/madd-4 gene in which UNC-29–RFP fluorescence was decreased at NMJs, and clusters were formed in extrasynaptic regions of the muscle cells (Fig. 1b and Extended Data Fig. 1a)5. This gene encodes three isoforms of an ADAMTS-like protein orthologous to the poorly characterized mammalian punctin-1/ADAMTS-like1 and punctin-2/ADAMTS-like3 proteins (Extended Data Fig. 1d, e)17, 18. ADAMTS-like proteins are secreted modular proteins of the extracellular matrix that contain domains similar to ADAMTS proteases but lack the metalloprotease and disintegrin amino-terminal domains characteristic of ADAM proteases6. MADD-4A and MADD-4C are the longest isoforms, differing by alternative splicing of a two-residue encoding exon (Extended Data Fig. 1a, b). MADD-4B is a shorter isoform corresponding to the carboxy-terminal part of MADD-4A and MADD-4C and probably generated by the use of an alternative promoter. In C. elegans, MADD-4B is secreted by motoneurons and serves as a guidance cue for the growth of the so-called muscle arms5. These muscle cell extensions grow during post-embryonic development to reach motoneuron axons and establish en passant synapses at the dorsal and ventral nerve cords (Fig. 1a)19. Each muscle arm receives both cholinergic and GABAergic inputs. We generated a molecular null allele of madd-4, namely kr270, by deleting the entire coding sequence of madd-4 (Extended Data Fig. 1a). By immunofluorescence staining we found that cholinergic boutons were properly formed in this mutant, and ultrastructural analysis revealed no defect of presynaptic differentiations or synaptic cleft morphology (Fig. 1c–h and Extended Data Figs 2a–f and 3). However, L-AChR aggregates were redistributed to extrasynaptic areas (Fig. 1c–h and Extended Data Fig. 2a–f). To test whether L-AChRs were properly inserted in the plasma membrane, we first stained surface L-AChRs in vivo by using a strain expressing L-AChRs carrying an extracellular Myc tag20, 21. Extrasynaptic L-AChRs were readily detected by fluorescent anti-Myc antibodies injected into the pseudocoelomic cavity of madd-4 mutants (Extended Data Fig. 2g, h). Second, we performed an electrophysiological analysis. Pressure ejection of levamisole onto voltage-clamped muscle cells elicited similar currents in the wild type and in madd-4 mutants (Fig. 1i), indicating that L-AChRs were fully functional. However, evoked currents triggered by motoneuron stimulation were strongly reduced (Fig. 1j), in agreement with the reduction of synaptic L-AChRs detected by UNC-29–RFP visualization and UNC-38 immunofluorescence staining. In C. elegans, cholinergic NMJs also contain homomeric N-AChRs (nicotine-sensitive AChRs) composed of the ACR-16 subunit22, 23. The distribution of N-AChR was similar to that of L-AChRs in madd-4 mutants (Extended Data Fig. 2i, j). In addition, the muscle GABAAR UNC-49 formed clusters that redistributed at extrasynaptic areas of muscle cells, in a similar manner to AChRs, whereas GABAergic boutons were properly formed in madd-4 mutants (Fig. 1k–p)24. Therefore madd-4 is required for the differentiation of postsynaptic domains independently from presynaptic differentiation at cholinergic and GABAergic NMJs. We previously demonstrated that the removal of either LEV-9 or LEV-10 scaffold protein leads to the complete disruption of the L-AChR clustering complex12, 13. Because extrasynaptic L-AChRs still formed clusters in madd-4 mutants, we tested whether these clusters remained associated with the scaffolding machinery. First, we observed that LEV-9 and LEV-10 were present in synaptic and extrasynaptic L-AChR clusters in madd-4 mutants (Extended Data Fig. 2k–p). Second, we genetically inactivated lev-9 and lev-10 in madd-4 mutants and observed that L-AChRs were no longer clustered (Extended Data Fig. 2q–s). Hence MADD-4 is not required for the assembly of the L-AChR clustering machinery itself but is necessary to recruit or stabilize L-AChR complexes at postsynaptic sites. To analyse the subcellular distribution of MADD-4 variants, we generated functional green fluorescent protein (GFP)-tagged reporters for MADD-4L long isoforms (which globally refers to MADD-4A and MADD-4C) and MADD-4B in the context of a rescuing fosmid construct (Extended Data Figs 1c and 4)25. First, we verified that MADD-4 isoforms were indeed secreted proteins as suggested by the presence of predicted signal peptides (Extended Data Figs 1d and 5a–f). Second, we observed that MADD-4L–GFP was juxtaposed to cholinergic terminals but not to GABAergic terminals, whereas MADD-4B–GFP was juxtaposed to both types of bouton (Fig. 2). These observations are consistent with the differential expression of madd-4L and madd-4B promoter fragments in excitatory motoneurons or in both types of motoneuron, respectively5. Third, we injected anti-GFP antibodies in vivo and specifically stained extracellular MADD-4L–GFP and MADD-4B–GFP at NMJs, indicating the secretion and retention of these proteins in the synaptic cleft (Extended Data Fig. 5g–l). Because MADD-4B was previously reported to require the kinesin UNC-104 to be properly secreted at the dorsal cord5, we analysed L-AChR and GABAAR distribution in unc-104 mutants and observed extrasynaptic receptor clusters as in madd-4 mutants (Extended Data Fig. 5m–p). To test whether the localization of MADD-4A–GFP and MADD-4B–GFP depended on AChRs, we analysed their distribution in an unc-29; acr-16 double mutant and found that they were properly localized at cholinergic NMJs (Extended Data Fig. 6a–d). In addition, MADD-4B–GFP was retained at GABAergic synapses in the absence of GABAAR (Extended Data Fig. 6e–h). MADD-4 isoforms are therefore secreted and retained at synapses independently from postsynaptic receptors, and probably serve as molecular landmarks to define the synaptic zone on the postsynaptic membrane. Because our previous observations were made in adult nematodes, the redistribution of L-AChR and GABAAR at extrasynaptic areas might have resulted from defective formation or defective maintenance of receptor clustering. Soon after hatching, clusters of L-AChRs and GABAARs spread outside the nerve cords in madd-4 mutant, in contrast with the clusters confined to NMJs in the wild type (Fig. 3a–h), indicating that madd-4 may be required early during synapse formation. Consistently, MADD-4L–GFP and MADD-4B–GFP were readily detected at the nerve cords at this stage (Fig. 3i, j). To test directly whether MADD-4 can induce receptor clustering, we expressed MADD-4A–GFP ectopically in muscle cells of wild-type animals. Large ectopic L-AChR clusters co-localized with ectopic MADD-4A–GFP punctae, whereas the intensity of synaptic clusters was greatly decreased, suggesting that MADD-4A overexpression at extrasynaptic sites outcompeted the normal complement of receptors at the synapse (Fig. 3k–m). These ectopic clusters could also form in the absence of MADD-4B (data not shown). In addition, ectopic expression of MADD-4A–GFP in the FLP sensory neurons triggered ectopic clustering of L-AChRs on the internal side of the muscle close to the anterior FLP process (Fig. 3n–p). Altogether, these data suggest that madd-4 has an early function during synaptogenesis and is able to instruct postsynaptic receptor clustering at the synapse. Given the differential localization of the short and long isoforms of MADD-4, we speculated that each isoform might have a specific function in the differentiation of excitatory and inhibitory postsynapses. We individually reintroduced each isoform driven by its own promoter in a madd-4 null mutant. Synaptic L-AChR content, estimated by measuring UNC-29–RFP fluorescence at the dorsal nerve cord, was reduced by 60% in a madd-4 null mutant. This decrease was fully rescued by MADD-4L expression, whereas MADD-4B had no rescuing activity (Fig. 4a). To analyse the contribution of each isoform further, we used mutations that specifically disrupt either the short or the long isoforms (referred to as madd-4B(0) and madd-4L(0), respectively; Fig. 4b and Extended Data Fig. 1a). Electrophysiological analysis indicated that the total amount of L-AChRs present at the muscle cell surface was similar to that in the wild type in both mutants (Fig. 4c). In contrast, L-AChR-dependent evoked currents were significantly reduced in madd-4L(0) mutants but were wild-type in madd-4B(0) mutants (Fig. 4d). Consistently, L-AChR localization was wild-type in madd-4B(0) mutants or when MADD-4L was expressed in the madd-4 null mutant (Extended Data Figs 7 and 4d–i, respectively). The two long isoforms A and C had similar L-AChR clustering activities (Extended Data Fig. 8a). Taken together, these results demonstrate that the long isoforms are necessary and sufficient for localizing L-AChR clusters at NMJs. Because MADD-4B is required for the guidance of postsynaptic muscle arms5, the mislocalization of L-AChRs in madd-4 mutants might have been a secondary consequence of development defects of the muscle arms. However, several lines of evidence demonstrate that MADD-4 controls L-AChR synaptic clustering independently of muscle arm growth. First, disruption of madd-4 causes the disappearance of L-AChR clusters at SAB synapses (Extended Data Fig. 2a–f), which are directly made en passant between SAB axons and muscle cell soma with no requirement for muscle arm formation4. Second, the madd-4L(0) mutation causes L-AChR localization defects as severe as those in the madd-4 null mutant, whereas muscle arms are wild-type (Extended Data Fig. 9). Third, the madd-4B(0) mutant is impaired in muscle arm development5 but it does not show defects in L-AChR localization or function (Fig. 4c, d and Extended Data Fig. 7a–f). We then analysed the localization of GABAARs in the absence of MADD-4 isoforms. Specific ablation of the short isoform caused a striking phenotype: GABAARs still assembled into synaptic clusters but were now present at cholinergic synapses (Fig. 5a–c, g–i). A similar redistribution was achieved by re-expressing MADD-4L using its own promoter or a cholinergic-specific promoter in the madd-4 null mutant (Extended Data Figs 4m–o and 8b–f). In contrast, GABAARs were properly clustered at GABAergic NMJs in madd-4L(0) mutants (Fig. 5d–f, j–l) or in transgenic lines expressing MADD-4B in madd-4 null mutants (Extended Data Fig. 4p–r). These striking results indicate, first, that the short isoform is essential for clustering GABAARs at GABAergic NMJs and second, that the long isoform might be able to cluster both AChRs and GABAARs irrespective of presynaptic identity. To test this latter hypothesis, we expressed MADD-4A in GABAergic motoneurons. L-AChRs were now present opposite GABAergic release sites and mixed with GABAARs as assessed by the strong co-localization of the two types of receptor (Fig. 5m–v and Extended Data Fig. 8l–n). These results raise an apparent paradox. Indeed, in wild-type animals the long isoforms are present only at cholinergic NMJs, yet GABAARs are excluded from these synapses. A simple model would propose that an interaction between the short and long isoforms at cholinergic junctions could inhibit the GABAAR clustering activity of the long isoforms. In agreement with this model, less GABAARs co-localized with L-AChRs at cholinergic NMJs when co-expressing both MADD-4A and MADD-4B in cholinergic neurons than when expressing the long isoform alone (Extended Data Fig. 8g–k, l–n). To test whether long and short isoforms can interact molecularly, we performed co-immunoprecipitation experiments in human embryonic kidney (HEK) cells. Results showed that MADD-4 isoforms can undergo homophilic and heterophilic interactions (Extended Data Fig. 10), which might account for the functional interactions observed in vivo. We have identified the ADAMTS-like Ce-Punctin gene as a critical organizer of postsynaptic domains at C. elegans neuromuscular junctions. The short and long Ce-Punctin isoforms are differentially expressed by cholinergic and GABAergic motoneurons. Long Ce-Punctin isoforms localize AChR clusters at excitatory NMJs, whereas the short Ce-Punctin isoform prevents the recruitment of GABARs to cholinergic synapses and promotes GABAR clustering at GABA synapses. In contrast with models of synaptic organization relying on trans-synaptic interactions of proteins that ultimately connect presynaptic and postsynaptic membranes1, 2, 3, our results suggest an alternative model that relies on the local release of a neurally secreted protein that acts as a molecular landmark triggering the clustering of specific receptors. This might be achieved through the activation of a signal-transducing receptor, as in the agrin/MuSK pathway at the mammalian NMJ26, 27. Alternatively, the long Ce-Punctin isoforms might directly recruit the L-AChR-associated scaffold through protein–protein interactions, because ADAMTS-like proteins are known to bind extracellular proteins such as fibrillin and latent transforming growth factor-β binding protein28, 29. The ability of ADAMTS-like proteins to organize the synapse was unknown until we characterized the phenotype of the Ce-punctin/madd-4 mutants. Because the ADAMTSL3/punctin-2 gene is expressed in the central nervous system (based on Allen Mouse Brain Atlas30) and was identified as a candidate gene for schizophrenia7, it will be interesting to test whether the synaptic functions of Ce-Punctin have been conserved during evolution. Strains were grown at 20 °C unless otherwise stated on NGM agar plates seeded with Escherichia coli OP50 as a food source31. The wild-type strain was C. elegans N2 Bristol. Cohorts of synchronized L1 larvae were obtained by collecting eggs at 20 °C for 1 h, transferring the eggs to 15 °C for 14 h and then to 20 °C until hatching. Freshly harvested L1 larvae were observed 2 h after hatching. Transgenic lines based on the rescue of lin-15(n765ts) were grown at 25 °C before immunofluorescence staining. The following mutant alleles and transgenes were used in this study: LGI: unc-29(x29), unc-29(kr208::tagRFP)15, madd-4(kr270), madd-4(kr249), madd-4(ok2862), madd-4(tr185)5, madd-4(ttTi103747), lev-10(kr26)12; LGII: unc-104(e1265); LGIII: unc-49(e407); LGIV: juIs1[Punc-25::snb-1–GFP]32; LGV: acr-16(ok789); LGX: lin-15(n765), lev-9(ox177), lev-9(kr184::T7)13; ljEx42 [Punc-38::unc-38-MYC-6xHIS-2 × Myc, rol-6]20, jaSi4[Pmyo-3::acr-16-GFP]33, trIs25 [pPRRF138.2(him-4p::MB::YFP)]5. The following transgenic lines were created for this study: (1) In unc-29(kr208::tagRFP) madd-4(kr249); lin-15(n765): krEx1028 [pBP31; pEKL15]; krEx1097, krEx1098 and krEx1099 [pBP8; pEKL15]; krEx1100, krEx1101 and krEx1102 [pBP11; pEKL15]; krEx1085, krEx1086 and krEx1087 [pMP9; pEKL15]; krEx1093 and krEx1094 [pBP23; pMP1; pEKL15]; krEx1039, krEx1135 and krEx1136 [pEKL15]; krEx1042, krEx1043 and krEx1044 [pBP23; pEKL15]; krEx1067 and krEx1068 [pBP35; pBP33; pEKL15]; krEx1069 and krEx1070 [pBP36; pBP33; pEKL15]; krEx1084 [pBP34; pBP33; pEKL15]; krEx1156 [pBP70; pRF4]. (2) In unc-29(kr208::tagRFP); lin-15(n765): krEx970 [Phim-4::mb-yfp; pEKL15]. The madd-4(kr270) molecular null allele was generated by using the Mos1-mediated deletion (MosDEL) technique as described16, 34. The entire coding sequence of madd-4, starting from the ATG of madd-4A and madd-4C, was replaced with the Caenorhabditis briggsae unc-119 wild-type gene. The pBP6 vector was injected as a rescue template into a strain containing the ttTi21487 Mos1 insertion, internal to the madd-4 gene. In pBP6, a 3-kilobase (kb) left madd-4 homology sequence and a 2-kb right homology sequence flank the unc-119 marker. The krSi4 allele encodes a madd-4A–GFP minigene and was generated by using the Mos1-mediated single-copy insertion (MosSCI) technique as described16, 35. The madd-4A–GFP minigene is composed of 2,652 base pairs (bp) of madd-4A promoter, a genomic DNA fragment containing the first 5 exons of madd-4A, a complementary DNA fragment corresponding to the last 12 exons, and the sequence of eGFP and the 3′ untranslated region (UTR) sequence of unc-54. The EG4322 strain was injected with pBP27 as a rescue template, a vector in which the madd-4A–GFP minigene was inserted into pCFJ151 by using XhoI and SdaI sites. The krSi2 allele was generated by MosSCI. It encodes a unc-49B-tagRFP minigene, composed of 4,070 bp of unc-49 promoter, a genomic DNA fragment containing the first four exons of unc-49, a cDNA fragment corresponding to the last eight exons of unc-49B isoform and the 3′ UTR sequence of unc-54. The tagRFP sequence was inserted in the TM3–TM4 intracellular loop of the UNC-49B subunit. The EG4322 strain was injected with the pTH22.1 vector, in which the unc-49B-tagRFP minigene fused with the 3′ UTR sequence of unc-54 was inserted into pCFJ151 by using SbfI and SpeI sites. Transformation was performed by microinjection of plasmid DNA into the gonad as described36. For generation of the fosmid-based lines, the pBP35 fosmid (or pBP36) was injected at 10 ng μl−1 with pBP33 (Punc-47::snb-1–GFP) at 30 ng μl−1, pEKL15 (lin15(+)) at 30 ng μl−1 and 1 kb ladder (New England Biolabs) up to 100 ng μl−1. The pBP34 fosmid was injected at 1 ng μl−1 with pBP33 (Punc-47::snb-1–GFP) at 30 ng μl−1, pEKL15 (lin15(+)) at 30 ng μl−1 and 1 kb ladder up to 100 ng μl−1. For tissue-specific expression of the isoforms of madd-4 or madd-4–GFP in cholinergic motoneurons, GABAergic motoneurons or muscle cells, pBP8, pBP11, pBP23, pMP1, pMP9 or pBP31 was injected at 10 ng μl−1 with pEKL15 (lin15(+)) at 30 ng μl−1 and 1 kb ladder up to 100 ng μl−1. pBP23 and pMP1 plasmids were also co-injected at 10 ng μl−1 each with pEKL15 (lin15(+)) at 30 ng μl−1 and 1 kb ladder up to 100 ng μl−1. pBP70 was injected at 50 ng μl−1 along with pRF4 at 50 ng μl−1. For labelling of muscle cells, animals were injected with a DNA mixture containing Phim-4::mb-yfp19 at 10 ng μl−1, pEKL15 (lin15(+)) at 30 ng μl−1 and 1 kb ladder up to 100 ng μl−1. For the generation of madd-4(kr270) by MosDEL16, 34, the pBP6 repair template vector was injected at 50 ng μl−1 with pJL43 (Mos transposase under a germline-specific promoter) at 50 ng μl−1, Prab-3::GFP (a pan-neuronal marker) at 15 ng μl−1 and Pmyo-2::GFP (a pharynx-specific marker) at 5 ng μl−1. For the generation of krSi4 by MosSCI16, 35, the pBP27 vector was injected at 50 ng μl−1 with pJL43 at 50 ng μl−1, Prab-3::GFP at 15 ng μl−1 and Pmyo-2::GFP at 5 ng μl−1. For the generation of krSi2 by MosSCI, the pHT22.1 vector was injected at 60 ng μl−1 with pJL43 at 50 ng μl−1, Prab-3::GFP at 15 ng μl−1 and Pmyo-2::GFP at 5 ng μl−1. We generated fosmids for tagging different isoforms of madd-4 with GFP, using the fosmid recombineering technique25. Specifically, we first added a GFP tag at the C terminus of madd-4, right after the last residue, using the WRM0626CA02 fosmid, which contained 37 kb of genomic sequences at the madd-4 locus, as a template. The recombination fragment was generated by amplification of GFP from pBP32 (a vector derived from pBALU1 (ref. 25) by introducing the S65T mutation in the GFP sequence) flanked by 200-bp madd-4 homology sequences on each side. In a second step, we recombineered the pBP34 fosmid to delete the first exon of madd-4A and madd-4C and to make a madd-4B–GFP reporter (pBP36) or to remove the first exon of madd-4B and to generate a fosmid that drives madd-4A–GFP and madd-4C–GFP expression (pBP35). To achieve these deletions, the recombination fragments were composed of the GalK cassette amplified from pBALU8* (ref. 25) and 100 bp of homology sequences to the pBP34 fosmid. The following vectors were generated by Gateway cloning (Invitrogen). They bear the cDNA of madd-4A and madd-4B, which were obtained by RT–PCR on mixed-stage nematodes RNA extracts. The GFP tags were inserted at the C-terminal end. pBP8: Punc-17::madd-4C; pBP11: Punc-17::madd-4A; pBP23: Punc-17::madd-4A–GFP; pMP1: Punc-17::madd-4B–GFP; pMP9: Punc-47::madd-4A–GFP; pBP31: Pmyo-3::madd-4A–GFP; pBP70: Pegl-46::madd-4A–GFP; pBP33: Punc-47::snb-1-3Xmyc. This reporter for GABA motoneuron presynapses was generated by replacing the GFP sequence of pJL35 (Punc-47::snb-1/vamp–GFP) by a 3 × Myc tag and further cloned using a Gateway cloning strategy (Invitrogen). In these vectors, the promoter fragments were given by pRH81 (unc-17 promoter, a gift from E. Jorgensen), pEGB06 (unc-47 promoter, a gift from E. Jorgensen) and pBP15 (myo-3 promoter, derived from pmyo-3[4-1], a gift from M. Hammarlund). The unc-54 3′ UTR ENTRY vector was a gift from M. Hammarlund. A 3.1-kb promoter region of egl-46 was amplified from genomic DNA37. For expression in HEK cells, madd-4A and madd-4B cDNAs were tagged at the C-terminal end with 3 × Myc or GFP tags and the fusion proteins were driven by the pCMV promoter in a pCDNA3.1 backbone (Invitrogen). The plasmids were as follows: pBP38: Pcmv::madd-4A–GFP; pBP39: Pcmv::madd-4A-3xmyc; pBP40: Pcmv::madd-4B–GFP; pBP41: Pcmv::madd-4B-3xmyc. All plasmid sequences are available from the authors on request. Electrophysiological methods were as described previously38, 39. L-AChR-dependent evoked currents were recorded in the presence of dihydro-β-erythroidine (5 μM), which selectively inhibits N-AChRs. The experimental sample size was estimated by using values from previous wild-type electrophysiology data, using a 90% confidence level of detecting a less than 0.05 significant difference in amplitudes. Mann–Whitney tests were performed to compare the different genotypes. Detection of L-AChR at the surface of muscle cells by antibody injection was performed as described21. Monoclonal anti-c-Myc-Cy3 antibodies (C6594; Sigma-Aldrich) and polyclonal anti-GFP-Alexa Fluor 555 antibodies (A-31851; Invitrogen) were used. Immunohistochemistry was performed as described13. In brief, C. elegans were freeze-cracked and processed through acetone/methanol fixation except for LEV-9-T7, for which paraformaldehyde fixation was used. A mix of primary antibodies or of secondary antibodies were incubated overnight at 4 °C. Primary antibodies were used at the following dilutions: anti-UNC-38, 1:500 (ref. 13); anti-UNC-49, 1:500 (ref. 40); anti-VAChT/UNC-17, 1:1,000 (ref. 12); monoclonal anti-GFP (JL-8; Clontech), 1:500; polyclonal rabbit anti-GFP (Molecular Probes A11122; Invitrogen), 1:500; monoclonal 9E10 anti-Myc (11667149001; Roche), 1:500. Secondary antibodies included Cy3-labelled goat anti-rabbit immunoglobulin G (IgG) used at 1:1,000 (Jackson ImmunoResearch Laboratories A10520; Invitrogen), Cy3-labelled goat anti-mouse IgG at 1:1,000 (Jackson ImmunoResearch Laboratories A10521; Invitrogen), Alexa 488–labelled goat anti-rabbit IgG (Molecular Probes A-11008, Invitrogen) at 1:500, Alexa 488–labelled goat anti-mouse IgG at 1:500 (Molecular Probes A-11001; Invitrogen), Dylight 649-conjugated goat anti-mouse IgG at 1:500 (115-495-146; Jackson ImmunoResearch Laboratories) and Dylight 649-conjugated goat anti-rabbit IgG at 1:500 (111-495-144; Jackson ImmunoResearch Laboratories). Immunofluorescence staining experiments were repeated independently at least three times. Animals were mounted on 2% agarose pads, anaesthetized with 5 μl of M9 buffer containing 100 mM sodium azide and examined with either a Leica 5000B microscope equipped with a spinning disk CSU10 (Yokogawa) and a Coolsnap HQ2 camera, or a Nikon Eclipse Ti equipped with a spinning disk CSUX1-A1 (Yokogawa) and an Evolve EMCCD camera. Image reconstruction and merges were obtained with Image J. All images shown are projections of Z-stacks 2–20 μm deep and, with the exception of Fig. 3 and Extended Data Figs 2g–j and 5a–f, the background was subtracted with the Subtract Background tool of Image J (rolling ball radius 50 pixels). To remove the autofluorescence of intestinal granules in L1 larvae, a projection of Z-stack images acquired in the green channel was subtracted from a projection of Z-stack images of L-AChR (unc-29(kr208::tagRFP)), acquired in the red channel with the Image Calculator tool in Image J (Fig. 3a, b). For pictures of MADD-4L–GFP and MADD-4B–GFP in L1 larvae (krEx1067 and krEx1069; Fig. 3i, j), the blue channel was used in a similar way to minimize the autofluorescence of intestinal granules. Quantification of synaptic L-AChR at the dorsal cord in the unc-29(kr208::tagRFP) knock-in background was achieved by following a previously published protocol, which was initially described for in vivo labelling with anti-Myc–Cy3 (Fig. 4a and Extended Data Fig. 8a)21. Fluorescence levels were calculated for the anteriormost 30-μm portion of the dorsal cord. Independent transgenic lines were analysed for each genetic background, on different days (two and three independent lines for Fig. 4a and Extended Data Fig. 8a, respectively). For each line, the normality of distribution was controlled by a Kolmogorov–Smirnov test. The lack of significant difference between data obtained from the independent transgenic lines was tested by two-tailed Student’s t-tests before pooling data together. Data are presented as a percentage of the mean fluorescence relative to that of control lines acquired on the same days. Eventually, two-tailed Student’s t-tests were performed to compare the data between different genotypes. To evaluate the level of co-localization between L-AChR and GABAAR, immunostaining using anti-UNC-49 antibody was performed in the unc-29(kr208::tagRFP) knock-in background. Anti-UNC-49 antibodies were revealed with a Dylight 649-conjugated secondary antibody. The fluorescence of tagRFP persisted on immunostaining treatment. In a first analysis, we generated one-dimensional projections of Z-stacks corresponding to a region of approximately 150 μm of the anterior dorsal cord by using Image J. The background was removed using the Subtract Background plugin of Image J (rolling ball radius 50 pixels). The fluorescence intensity along the cord was evaluated with the Plot Profile plugin. For each channel, the values were normalized to the value of maximal intensity. The corresponding plots are presented in Fig. 5q, v and Extended Data Fig. 8f, k. The distribution of fluorescence intensity in each field was compared by using the mean of the squares of Pearson’s coefficient’s (r2). Analysis of variance (ANOVA) tests were performed to analyse data obtained in the different genetic backgrounds. The plot profiles shown in Fig. 2d, h, l, p and Extended Data Fig. 6h were generated with the same tools. In the analysis presented in Extended Data Fig. 8l–n, the co-localization of synapses containing GABAAR and L-AChR was examined by identifying the peak of fluorescence in one-dimensional profiles of fluorescence in each colour. To avoid detecting the same clusters twice, the peaks used were the local maxima in a moving window 2.5 μm wide. Only large peaks (that is, with an intensity 1 s.d. above the average fluorescence) were considered. The density of L-AChR clusters around GABAAR clusters was then obtained by constructing the histogram of the distances between all pairs of synapses of different types. For statistical comparison, the amplitude of the peak density above the baseline density was compared across genotypes by ANOVA followed by a pairwise Student’s test (with Holm adjustments to the P values for repeated comparisons). The statistical analysis was performed blind to the genotype. High-pressure freezing, freeze substitution, electron microscopy section preparations and electron tomography were performed as described previously41. The HEK293 cells were maintained in DMEM medium (Invitrogen) with 10% inactive bovine fetal serum (Invitrogen). The cells were transiently transfected with indicated constructions (pBP38, pBP39, pBP40 and pBP41) by using jetPRIME transfection reagents (Polyplus). The cell culture supernatants were harvested at 3 days after transfection and used to perform immunoprecipitation after centrifugation to remove the cell debris (13,000 r.p.m. for 10 min at 4 °C). The cells were lysed with ice-cold immunoprecipitation buffer (IPB; 50 mM HEPES pH 7.7, 100 mM NaCl, 50 mM KCl, 2 mM MgCl2, 1 mM EDTA, 1 mg ml−1 pepstatin, 1 mg ml−1 leupeptin, 2 mg ml−1 aprotinin, 1 mM phenylmethylsulphonyl fluoride, one tablet of protease inhibitor cocktail per 10 ml of buffer) containing 1% Triton X-100 on ice for 60 min, then centrifuged at 13,000 r.p.m. for 10 min at 4 °C. Both the cell lysates and cell culture supernatants were pooled together and the mixture was diluted with IPB to a final concentration of 0.2% (w/v) Triton X-100. Further procedure followed a published protocol21. In brief, the prewashed 1:1 slurry of 50 μl of anti-GFP-Trap-A beads was added and incubated overnight at 4 °C with gentle rotation. The beads were collected by centrifugation at 1,000g for 3 min at 4 °C and washed once with IPB containing 0.2% Triton X-100, and twice with washing buffer (50 mM HEPES pH 7.7, 50 mM NaCl) containing 0.2% Triton X-100. The immunoprecipitated proteins were eluted in Laemmli buffer with 2-mercaptoethanol, and analysed by western blotting after being boiled for 10 min at 95 °C. The primary anti-Myc polyclonal antibody (Gene Tex) was used at a 1:10,000 dilution, and horseradish peroxidase-conjugated goat anti-rabbit (K4002; DAKO) was used as secondary antibody at 1:50 dilution. The images of blotting membranes were captured by Bio-Rad GelDoc XR+ with Image Lab software. Download references We thank C. Lena for expert assistance with analysis of postsynaptic receptor distributions; A. Boyanov for whole genome sequencing; L. Briseno-Roa for technical advice; T. Boulin and M. Jospin for critical reading of the manuscript; M. J. Caron for suggestions on the figure display; A. Valfort, H. Gendrot, P. Muller and B. Mathieu for technical help; J. Rand for the anti-UNC-17 antibodies; M. Hammarlund and E. Jorgensen for plasmids; and P. Roy, the Caenorhabditis Genetic Center (which is funded by National Institutes of Health Office of Research Infrastructure Programs, P40 OD010440) and the International C. elegans Gene Knockout Consortium for strains. B.P.-L. was supported by the Association Française contre les Myopathies; H.T. by the Fondation pour la Recherche Medicale and by the Neuropole de Recherche Francilien; M.P. by the Neuropole de Recherche Francilien; H.Z. by the Fondation Pierre-Gilles de Gennes; and C.S. by a Human Frontier Science Program Long-Term Fellowship. This work was funded by the Agence Nationale de la Recherche (ANR-2010-BLAN-1618-01 and ANR-11-BSV4-019), the Fondation pour la Recherche Medicale (‘Equipe FRM’ grant to J.-L.B.) and the Association Française contre les Myopathies (J.-L.B.).
更多查看译文
关键词
Molecular neuroscience
AI 理解论文
溯源树
样例
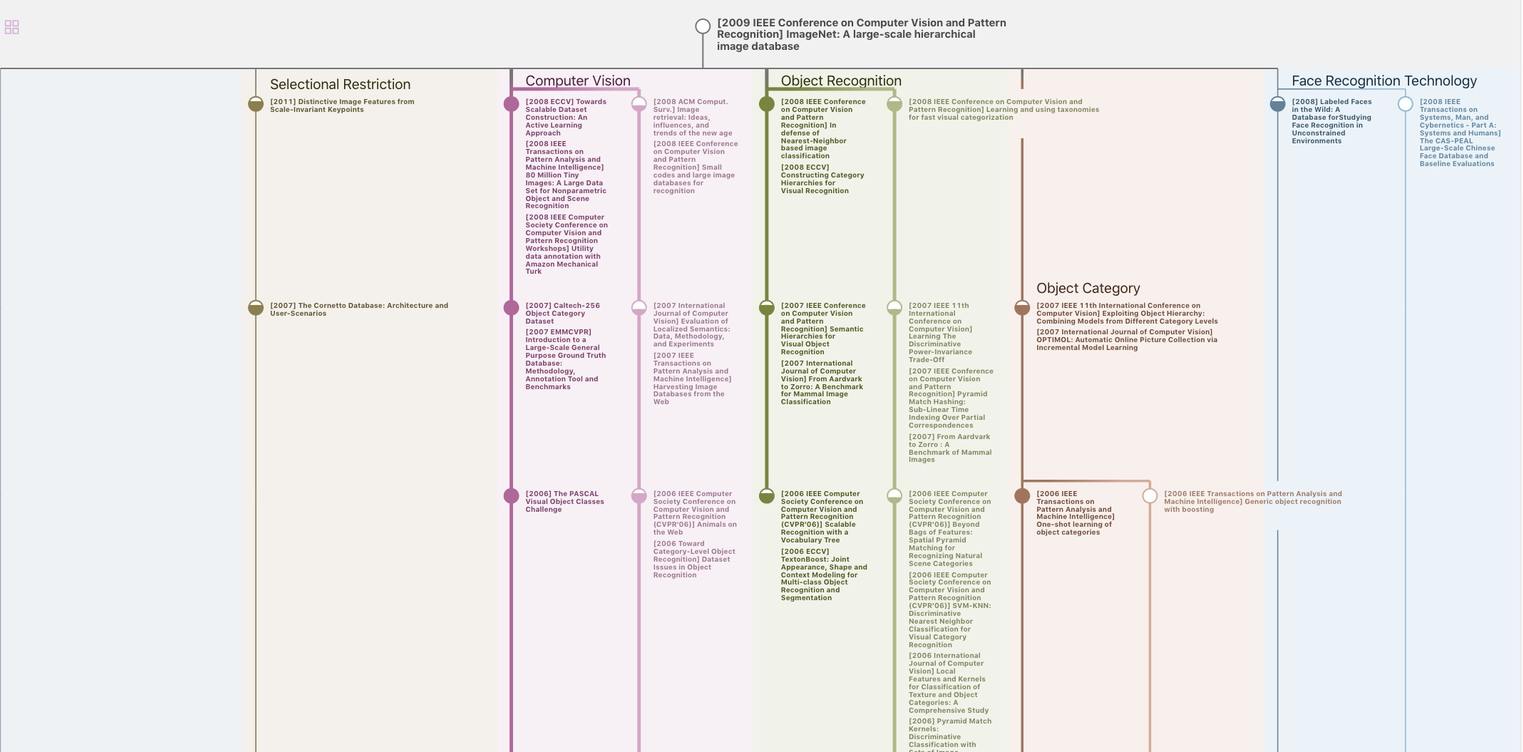
生成溯源树,研究论文发展脉络
Chat Paper
正在生成论文摘要