Quantifying the effect of localized depressurization on a deep underground orebody at the mcarthur river mine through cross hole hydraulic testing and groundwater modeling
semanticscholar(2012)
摘要
The McArthur River mine in northern Saskatchewan is the largest single producer of uranium in the world. Most of the ore is extracted by raisebore mining methods at depths of 530 to 600 m below ground surface where pore pressures in the fractured host sandstone and gneiss are on the order of 5 MPa. Currently, ground freezing is used to isolate the ore from ground-water sources. Localized depressurising of the freezing drifts is being considered to increase their ground-stability. Cross-hole flow and shut-in tests in eight NQ-size coreholes were conducted in the basement rock that is adjacent to a fault contact with the overlying 500 m thick sandstone unit. The hydrogeologic parameters of basement rock in the vicinity of a freezing drift were obtained. A 15% to 25% reduction of pore pressure over a 25 m distance was observed within a three hour test period. A detailed three-dimensional ground-water flow model was constructed to replicate the pore pressure measured in the coreholes. The pore pressure distribution simulated from the model provides the hydrogeologic input for geotechnical engineers to evaluate ground-stability and assess whether additional active depressurising should be conducted. INTRODUCTION The McArthur River mine, located in the southeastern part of the Athabasca Basin in northern Saskatchewan, Canada (Figure 1), is the largest single producer of uranium in the world. Most of the ore is extracted by raisebore mining methods at depths of 530 to 600 m below ground surface where pore pressures in the adjacent fractured sandstone are in the range of 5 MPa. Currently, ground freezing is used to isolate the ore from this high pressure ground-water. However, this methodology only enables ore to be extracted from the lower portion of the Zone 4 ore body (Figure 2) without additional freezing and utilizing the boxhole boring method. The potential of ground failure due to the high water pressure and low rock strength currently presents a challenge to extraction of a significant portion of the ore in the upper part of the Zone 4 ore body (Figure 2). Figure 1– Location of McArthur River mine Pre-mining depressurising of the entire ore body was initially considered as a method to decrease the risk associated with mining near the 5 MPa water pressure and increasing the amount of ore that can be extracted by the mining operation. The challenge is to depressurise the high-grade ore bodies -which are of relatively small lateral extent -without propagating a significant amount of drawdown to the surface where impacts on surface-water resources and associated aquatic habitat would be significant environmental issues. Another important issue predicted by the model was the volume of water that would need to be continuously discharged to achieve large scale depressurisation. In this environment, the volume of discharge was considered to be problematic (Liu et al, 2008). As an alternative to the mine-scale depressurisation, localized depressurising of the freezing drifts is being considered to increase their ground-stability. A preliminary program was designed and implemented to evaluate the effectiveness of local depressurisation of freezing drift. Cross-hole flow and shut-in tests were conducted in eight holes drill from the basement rock. A detailed groundwater flow model was conducted to simulate the reduction of pore pressure around the drifts as the result of the local depressurisation. FIELD AND NUMERICAL INVESTIGATION Basic Hydrogeology The upper bedrock at the McArthur River mine site consists of 480 to 560 m of sandstones of the Athabasca Group which unconformably overlie crystalline Archean and Aphebian basement rocks. The mineralisation being exploited at the mine is associated with a major thrust fault known as the P2 fault (Figure 2) where the majority of the mineralisation occurs along the southeast-dipping thrust at the contact between the Athabasca sandstones and underlying basement rocks in a series of discontinuous ore bodies (Figure 3). Figure 2 – Cross section of stratigraphy and ore body Figure 3 – Base map of mine area There are six major hydrostratigraphic units at the McArthur River mine including, from the stratigraphically highest to the lowest: post-glacial overburden, sandstone, fanglomerate with a basal paleo-weathered zone, an unconformity, the mineralized zone, and the basement rock. The measured horizontal hydraulic conductivity (Kh) values, as well as the geometric mean value, from packer testing conducted prior to shaft sinking are shown on Figure 4. These data show that the Kh of the sandstone unit is at least one order of magnitude greater than the basement rock. Figure 4 – Hydraulic conductivity vs. depth in sandstone Field Testing 10 coreholes were drilled from the face of the 7860N freeze drift. Figure 5 shows the plan view of these test locations. Figure 5 – Base map of McArthur River mine Figure 6 shows the collar locations of the 10 coreholes, the projected corehole trajectories, and the distances from the drift face to the end of each core holes (the “y” distance along azimuth 270o. Of the 10 coreholes, seven pass below the planned 7300 E drift and three pass above it (Figure 7). All the coreholes are NQ-size and have HQ-size surface casing grouted into the basement rock for about 6 m. All coreholes were connected to a drain manifold and installed with ball valves and hoses for flow and pressure measurement. A small diameter nylon tube was connected to each corehole valve on the drain manifold and to one of the inlet fittings on the pressure measuring panel. Valves within this panel allowed sequential measurement of pressures in each corehole by a high precision digital pressure gauge. Figure 6 – Corehole locations and hydraulic conductivity values from flow and shut-in tests from face of 7860N freeze drift Figure 7 – Pore pressure distribution in cross section looking north, 7860Nl Both flow and shut-in tests were conducted on each corehole for duration of about 200 to 380 minutes. The hydraulic conductivity (K) values from the flow tests were calculated using the JacobLohman method and the shut-in tests were evaluated using the Cooper-Jacob method [1]. The derived K values from the testing are summarized on Figure 6 Detailed Groundwater Flow Model Simulations Model Description Based on the results from the field testing, detailed three dimensional groundwater flow model was built to (1) represent the hydrogeologic conditions and mine workings in the vicinity of the drift, (2) replicate the hydraulic head response to the flow test of selected corehole, and (3) predict the pore pressure distribution with the current configuration of coreholes as input to geotechnical analysis. Figures 8 and 9 show the model domain in plan view and cross section, respectively. Note that elevations are referred to in mine levels which are true elevations in mamsl plau 1,000 m. The area of the model domain is about 120 m x 120 m. Its top is at 50 mamsl (the nominal 1050 mine level) and its bottom is at -50 mamsl (950 mine level). The model domain was discretised with 33,620 nodes, 60,800 elements, and 20 layers. To represent the geometry of the drifts, coreholes, and hydrogeology in the test area, a grid size of as small as 2 m in both the horizontal and vertical directions has been used in the model. The hydraulic properties assigned to each hydrogeologic unit are summarised on Figure 9. Figure 8 – Map view of model domain, geology represented, and hydraulic boundary conditions at nominal 1006 mine level Figure 9 – Model domain, geology represented, and hydraulic boundary conditions along Cross-Section A-A' The hydraulic boundary conditions assigned were: 1) A specified head of 510 mamsl at the top of the sandstone layer; this assumes that the relatively small amount of free drainage from the coreholes will have no significant effect on groundwater conditions in the sandstone, a major “aquifer”; 2) A similar specified head of 510 mamsl in the sandstone on the west boundary of the model; 3) Drain nodes with a head equal to elevation (i.e., h = z) for all nodes representing existing mine workings; and 4) No-flow conditions to all lateral model boundaries in the basement rock with the exception of the drain nodes assigned to the mine workings. For a hydrogeologic control volume with the size of this model, most of the ground-water flow would be controlled by fractures. However, to adequately represent such fractures, a well-defined discrete fracture network (DFN) would be required. This preliminary model assumes that each rock unit can be reasonably represented as an equivalent (albeit anisotropic) porous media.
更多查看译文
AI 理解论文
溯源树
样例
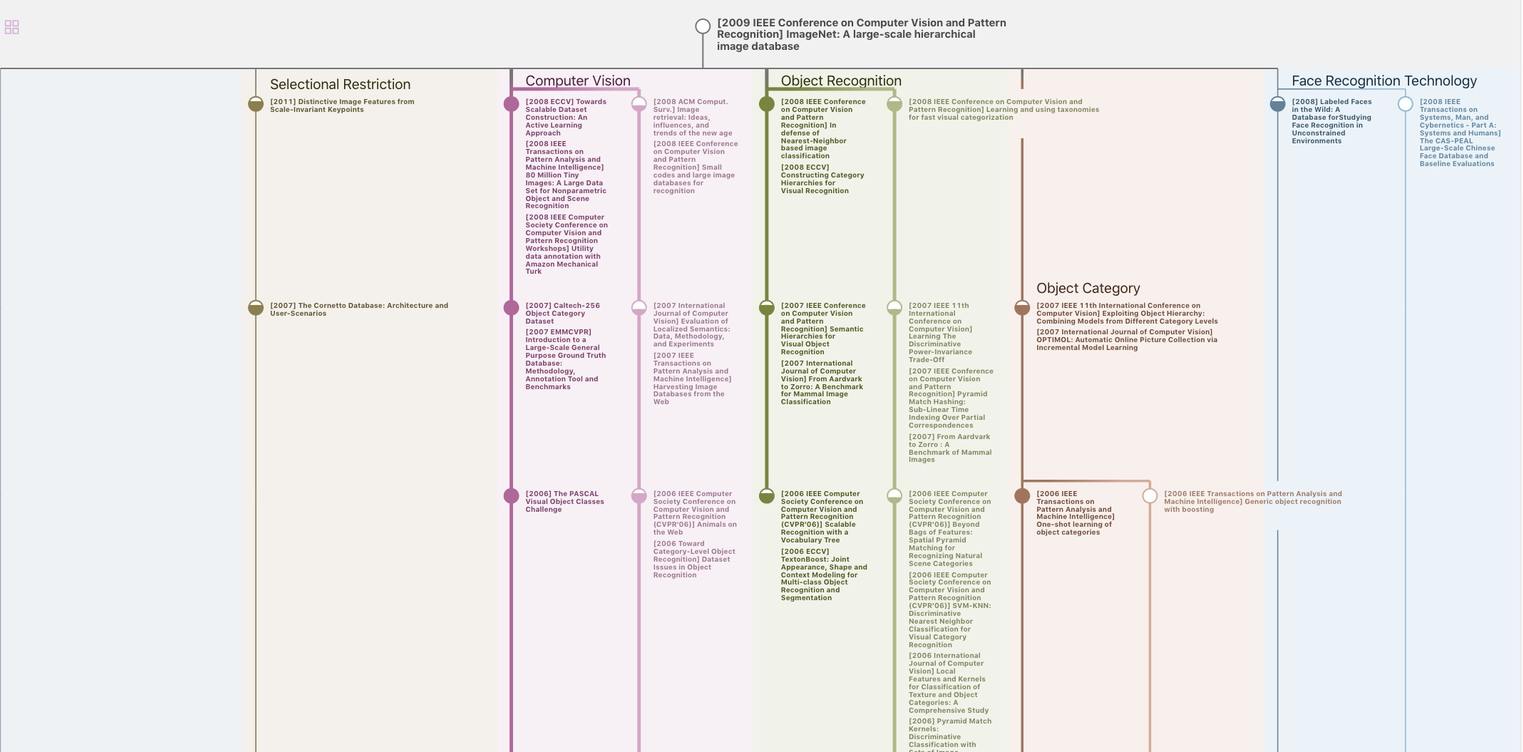
生成溯源树,研究论文发展脉络
Chat Paper
正在生成论文摘要