H E ] 2 1 O ct 2 00 9 Evidence for Proton-Dominated Cosmic Ray Composition above 1 . 6
semanticscholar(2009)
摘要
We report studies of ultra-high energy cosmic ray composition via analysis of depth of airshower maximum (Xmax), for airshower events collected by the High Resolution Fly’s Eye (HiRes) observatory. The HiRes data are consistent with a predominantly protonic composition of cosmic rays at energies above 1.6 EeV (10 eV), when interpreted via the QGSJET01 and QGSJET-II high-energy hadronic interaction models. We measure a mean Xmax at 10 EeV of 770.2 ± 2.1(stat.) ± 4.2(syst.) g/cm, and an elongation rate dXmax/d(log(E)) of 47.5 ± 5.9(stat.)± 2.8(syst.) g/cm/decade. These measurements constrain models in which the galacticto-extragalactic transition is the cause of the energy spectrum “ankle” at 4 × 10 eV. PACS numbers: 98.70.Sa, 95.85.Ry, 96.50.sb, 96.50.sd ∗Corresponding author: belz@physics.utah.edu The observation of a break in the cosmic ray energy spectrum at approximately 6 × 10 eV [1, 2] provides strong evidence that the highest energy cosmic rays are both extragalactic and protonic [3, 4]. Direct evidence for a proton-dominated composition from airshower data would lend further support to this model, as would the observation of a transition from (heavy) galactic to (light) extragalactic cosmic rays at lower energies. A second feature, the “ankle” of the energy spectrum at 4×10 eV may be indicative of this galactic to extragalactic transition or it may further strengthen the model in which the end of the cosmic ray spectrum is shaped by interactions with the cosmic microwave background [5]. Composition studies can provide decisive evidence in the choice between interpretations. An important clue to chemical composition which is accessible to air fluorescence observatories is the depth of shower maximum Xmax of cosmic ray induced extensive airshowers. Simple arguments [6, 7] show that the average value of airshower maximum will depend logarithmically on the primary energy and atomic mass, and that the elongation rate d /d logE will be constant for unchanging primary compositions. Further, to first order, a nucleus-induced shower may be thought of as a superposition of showers induced by single nucleons. Therefore due to averaging effects we also expect the width of the Xmax distribution at a given energy to be sensitive to the atomic mass of the primary. The two fluorescence observatories of the High-Resolution Fly’s Eye collected data in stereoscopic mode between December 1999 and April 2006. Located on the U.S. Army Dugway Proving Ground in Utah, at a mean elevation of 1,575 m MSL, a mean latitude of 40.16◦ N, and separated by 12.6 km, the observatories operated on clear moonless nights. Each detector consisted of an array of telescope modules, each module included a mirror of 3.7 m effective area which focused UV light from airshowers on a 16 × 16 photomultiplier tube (PMT) camera. The field of view of each PMT subtended a one degree diameter cone of the sky. The HiRes-I detector covered nearly 360◦ in azimuth, 3◦–17◦ in elevation and was read out by means of sample-and-hold electronics, while the HiRes-II detector covered 3◦–31◦ in elevation and was read out by a custom FADC system [8]. The calibration of the HiRes telescope modules has been described previously [9]. A portable Xenon flash lamp (∼ 0.5% stability) was used to illuminate each mirror monthly. Between Xenon runs, night-to-night calibrations were performed using YAG laser light delivered to the PMT clusters via optical fiber. A pulsed nitrogen laser was fired into the atmosphere from various locations within 3.5 km of the two detector sites. An overall accuracy of ∼ 10% RMS is achieved in the HiRes photometric scale. Steerable lasers fired patterns of shots which covered the aperture of the HiRes fluorescence detectors, in order to monitor UV attenuation in the atmosphere. The vertical aerosol optical depth (VAOD) was measured to be 0.04(mean) ± 0.02(RMS), corresponding to a mean correction of ∼15% upward in energy for an event 25 km distant from the observatory. In the present analysis, the steerable laser measurements were used to compile an hourly atmospheric database and hence allow hourly tuning of the atmospheric parameters. A mirror trigger was initiated if a sufficient number of PMTs were in temporal and spatial coincidence, then a stereo data set was obtained by the time-matching of HiRes-I and HiResII triggers. Geometrical reconstruction of stereo events proceeded by determination of the shower-detector plane from each HiRes site, the intersection of these two planes was taken to be the shower core trajectory. The resolution in the shower zenith angle is 0.6◦, and the resolution in Rp (distance of closest approach to the detector) is 1.2%. Hit information from multiple tubes in the HiRes-II data only are sorted into discrete time bins. In each bin, FADC signals are converted to a number of photoelectrons Npe, then adjusted for the effective area of each bin as determined by ray tracing. The geometry of the shower and the atmospheric databases are used to determine the atmospheric slant depth X for each shower bin. Shower segments that have emission angles within 5◦ of a bin’s pointing direction are flagged and not used for fitting due to excessive Cherenkov light contamination. The Npe profile is then converted to a profile of the fluorescence light at the shower by a routine that simulates the light production and propagation through the atmosphere, and subtracts the Cherenkov contribution. The intensity of fluorescence light emitted from an airshower is proportional to the total ionization energy deposited by the charged particles in the shower [10]. We use the average fluorescence yield of several groups [11, 12, 13] and the spectral distribution given by Ref. [14], along with the average dE/dX determined from CORSIKA simulations [15] to determine the number of charged particles in the shower as a function of slant depth. The shower profile is then fit to a Gaussian function of the age parameter s(X) = 3X/(X+ 2Xmax) in order to determine the airshower energy and Xmax. (Alternatively fitting by the Gaisser-Hillas parametrization [16] had little overall effect on the analyses and conclusions presented here.) Further details of the reconstruction used in this analysis are contained in Ref. [17]. FIG. 1: Comparison of current HiRes stereo results with results from the HiResprototype/MIA hybrid [21]. Also included are QGSJET01 (dashed) and QGSJET-II (solid) predictions for pure-proton and pure iron compositions. Ultra-high energy cosmic ray composition studies require a detailed comparison of data with the predictions of airshower models and are hence model dependent. In practice, these models are airshower Monte Carlo simulations. In order to completely understand the effects of the geometrical aperture of the detector, HiRes therefore applies a full detector simulation to the airshower Monte Carlo events. HiRes uses a library of 32,000 (20,000) simulated protonand iron-induced airshowers generated by the CORSIKA 6.003 (6.501) [15] package, using the QGSJET01 [18] (QGSJETII [19]) hadronic interaction models and the EGS4 [20] electromagnetic interaction driver. Each shower is sampled at 205 points along its development, recording the number of particles at each slant depth. Airshower longitudinal development is fit to a Gaussian-in-Age (GIA) functional form, and its as-thrown Xmax is recorded. The mean Xmax as a function of energy, for airshowers in the HiRes shower library is shown in Figure 1. Detector simulation proceeds by drawing an event from the shower library, assigning it a random core location and zenith and azimuthal angles and determining if it can trigger the detector. The number of charged particles at many discrete points along the shower is then determined, and fluorescence light is then propagated from the shower to the detector. Light attenuation by the atmosphere is realistically simulated by using an hourly database describing the measured aerosol content, temperature, and pressure. Ray tracing is performed to determine which phototubes actually see the light, allowing for photocathode response and inactive space between PMTs. An electronics simulation then determines the pulse height and time for each tube for HiRes-I electronics and forms a FADC waveform for HiRes-II electronics. For all tubes the channel gains, DAC settings, thresholds and channel variances are simulated by using hourly database information. The same trigger algorithms used in hardware are simulated to determine if either detector triggers in monocular mode. If an event is triggered, the simulated data is written to disk in the identical format as real data, thereby allowing the study of Monte Carlo events by the same analysis chain. The HiRes Monte Carlo data set contains approximately 20 times the number of reconstructed stereo events as the data. The major challenge in studying cosmic ray composition by the technique lies in understanding the systematic biases that occur during reconstruction and event selection. Low-energy showers which are nearby the detector may reach Xmax above the field of view of the mirrors, thus biasing a data set towards deeper showers. High-energy showers with small zenith angles are likely to reach maximum below the field of view of the mirrors, resulting in a bias towards shallow showers. It is useful to divide the types of biases which can occur into two types, called “acceptance biases” and “reconstruction biases”. Acceptance biases are due to events which fail reconstruction altogether, including detector triggering and event selection effects. Reconstruction biases are due to events which are successfully reconstructed, but with the wrong Xmax. The strategy in the following analysis is to choose event selection cuts which minimize the reconstruction bias, and make the acceptance bias as independent of energy as possible. After geometrical reconstruction and fitting of the shower p
更多查看译文
AI 理解论文
溯源树
样例
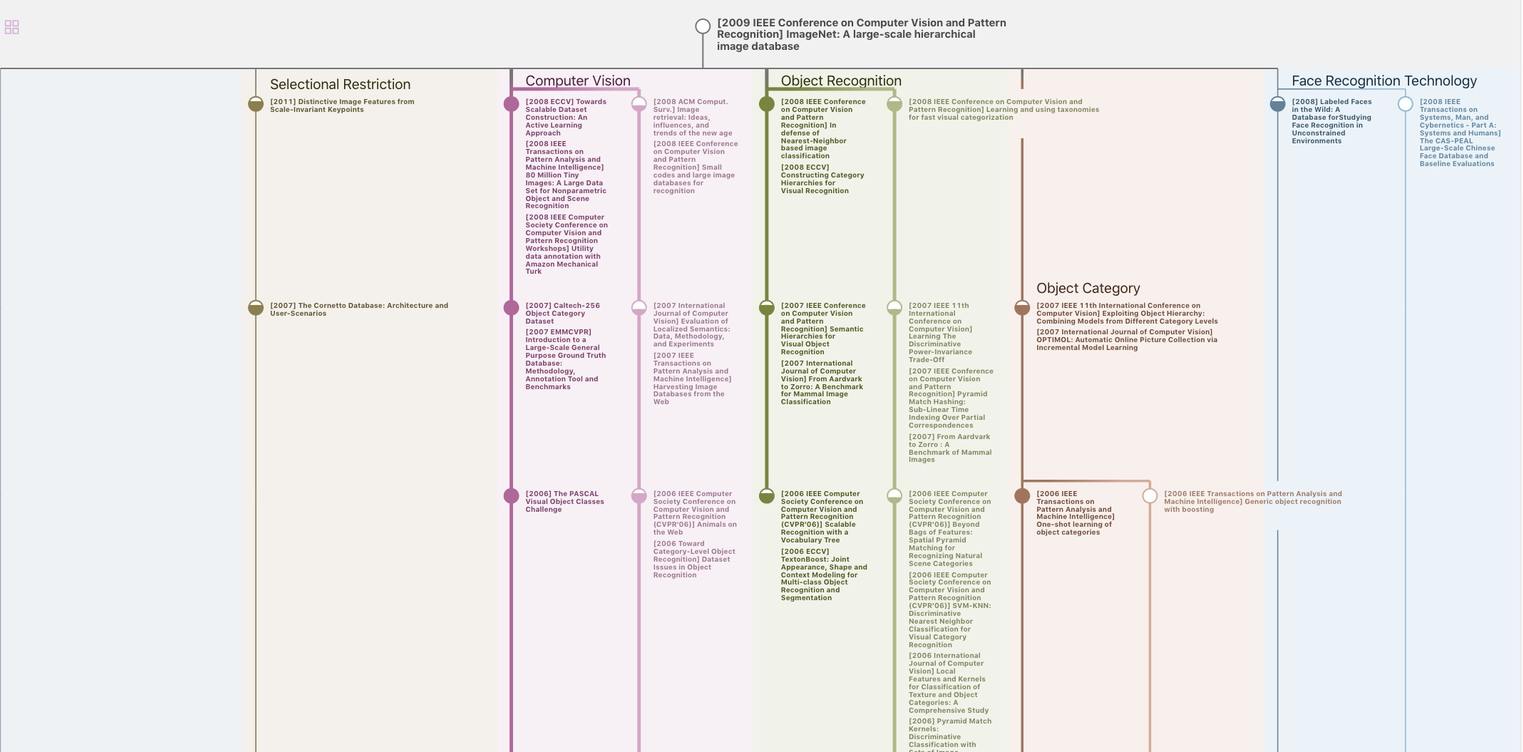
生成溯源树,研究论文发展脉络
Chat Paper
正在生成论文摘要