H+ -Driven Increase in CO2 Uptake and Decrease in HCO3− Uptake Explain Coccolithophores' Acclimation Responses to Ocean Acidification
Limnology and Oceanography(2016)
摘要
Limnology and OceanographyVolume 61, Issue 6 p. 2045-2057 ArticleOpen Access H+-driven increase in CO2 uptake and decrease in uptake explain coccolithophores' acclimation responses to ocean acidification Dorothee M. Kottmeier, Corresponding Author Dorothee M. Kottmeier Dorothee.Kottmeier@awi.de Department of Marine Biogeosciences, Alfred Wegener Institute, Helmholtz Centre for Polar and Marine Research, Bremerhaven, GermanyCorrespondence: Dorothee.Kottmeier@awi.deSearch for more papers by this authorSebastian D. Rokitta, Sebastian D. Rokitta Department of Marine Biogeosciences, Alfred Wegener Institute, Helmholtz Centre for Polar and Marine Research, Bremerhaven, GermanySearch for more papers by this authorBjörn Rost, Björn Rost Department of Marine Biogeosciences, Alfred Wegener Institute, Helmholtz Centre for Polar and Marine Research, Bremerhaven, GermanySearch for more papers by this author Dorothee M. Kottmeier, Corresponding Author Dorothee M. Kottmeier Dorothee.Kottmeier@awi.de Department of Marine Biogeosciences, Alfred Wegener Institute, Helmholtz Centre for Polar and Marine Research, Bremerhaven, GermanyCorrespondence: Dorothee.Kottmeier@awi.deSearch for more papers by this authorSebastian D. Rokitta, Sebastian D. Rokitta Department of Marine Biogeosciences, Alfred Wegener Institute, Helmholtz Centre for Polar and Marine Research, Bremerhaven, GermanySearch for more papers by this authorBjörn Rost, Björn Rost Department of Marine Biogeosciences, Alfred Wegener Institute, Helmholtz Centre for Polar and Marine Research, Bremerhaven, GermanySearch for more papers by this author First published: 15 July 2016 https://doi.org/10.1002/lno.10352Citations: 30AboutSectionsPDF ToolsRequest permissionExport citationAdd to favoritesTrack citation ShareShare Give accessShare full text accessShare full-text accessPlease review our Terms and Conditions of Use and check box below to share full-text version of article.I have read and accept the Wiley Online Library Terms and Conditions of UseShareable LinkUse the link below to share a full-text version of this article with your friends and colleagues. Learn more.Copy URL Share a linkShare onFacebookTwitterLinkedInRedditWechat Abstract Recent ocean acidification (OA) studies revealed that seawater [H+] rather than [CO2] or [ ] regulate short-term responses in carbon fluxes of Emiliania huxleyi. Here, we investigated whether acclimation to altered carbonate chemistry modulates this regulation pattern and how the carbon supply for calcification is affected by carbonate chemistry. We acclimated E. huxleyi to present-day (ambient [CO2], [ ], and pH) and OA conditions (high [CO2], ambient [ ], low pH). To differentiate between the CO2 and pH/H+ effects, we also acclimated cells to carbonation (high [CO2] and [ ], ambient pH) and acidification (ambient [CO2], low [ ], and pH). Under these conditions, growth, production of particulate inorganic and organic carbon, as well as carbon and oxygen fluxes were measured. Under carbonation, photosynthesis and calcification were stimulated due to additional uptake, whereas growth was unaffected. Such stimulatory effects are not apparent after short-term carbonation, indicating that cells adjusted their carbon acquisition during acclimation. Being driven by [ ], these regulations can, however, not explain typical OA effects. Under acidification and OA, photosynthesis stayed constant, whereas calcification and growth decreased. Similar to the short-term responses toward high [H+], CO2 uptake significantly increased, but uptake decreased. This antagonistic regulation in CO2 and uptake can explain why photosynthesis, being able to use CO2 and , often benefits from OA, whereas calcification, being mostly dependent on , often decreases. We identified H+ as prime driver of coccolithophores' acclimation responses toward OA. Acidified conditions seem to put metabolic burdens on the cells that result in decreased growth. Emiliania huxleyi is the Earth's most dominant pelagic calcifier and known to be well adapted to shallow mixed-layer depths with high irradiances (Nanninga and Tyrrell 1996; Raitsos et al. 2006). Under these conditions, the species is able to form large monospecific blooms with cell concentrations of up to 10 × 107 cells L−1 (Holligan et al. 1993; Tyrrell and Merico 2004). In the process of calcification, precipitates intracellularly with Ca2+ to form CaCO3, leading to reduced seawater levels and alkalinity. This production of particulate inorganic carbon (PIC) furthermore increases the partial pressure of carbon dioxide (pCO2) of seawater and thereby counteracts the effect of photosynthetic production of particulate organic carbon (POC). The relative strength of calcification vs. photosynthesis therefore influences the biogeochemical CO2 fluxes on regional and global scales (Broecker and Peng 1987; Rost and Riebesell 2004). In the last decades, E. huxleyi has become an important model organism, especially because of its high sensitivity toward ocean acidification (OA; Raven and Crawfurd 2012; Read et al. 2013; Meyer and Riebesell 2015). This term describes the strong increase in CO2 and the slight increase in HCO3 levels (their sum is referred to as carbonation) as well as the decrease in levels and pH (the latter corresponds to an increase in [H+] and is referred to as acidification), which result from the oceanic uptake of anthropogenic CO2 (Wolf-Gladrow et al. 1999; Caldeira and Wickett 2003). A large number of laboratory and field studies on E. huxleyi and other coccolithophores found that OA leads to unaffected or stimulated photosynthesis, with impaired or unaffected calcification and growth, typically leading to decreased PIC: POC ratios (Raven and Crawfurd 2012; Kroeker et al. 2013; Meyer and Riebesell 2015). These responses can yet vary in magnitude, depending on genetic predisposition and other environmental boundary conditions such as light, temperature, or nutrient status (Zondervan 2007; Langer et al. 2009; Lefebvre et al. 2012; Rokitta and Rost 2012; Sett et al. 2014; Xu and Gao 2015). In first attempts to identify the chemical drivers of typical OA responses, E. huxleyi was acclimated to decoupled carbonate chemistry, under which carbonation and acidification effects could be distinguished (Bach et al. 2011, 2013). These acclimation studies revealed that POC and PIC production are both stimulated by carbonation, but are reduced when cells are acclimated to acidification. The antagonistic regulation of PIC production by carbonation and acidification was also indicated by a study of Fukuda et al. (2014), who showed that calcification is reduced under high [H+], but that this reduction can be overcome by additional availability. A recent study investigated the mechanisms underlying short-term carbonation and acidification responses of present-day acclimated E. huxleyi by means of membrane-inlet mass spectrometry (Kottmeier et al. 2016). In this study, photosynthetic fluxes of E. huxleyi were shown to be relatively insensitive toward abrupt increases in CO2 and levels, i.e., when being exposed to carbonation for time scales of seconds to minutes. The fluxes were, however, very sensitive toward abrupt increases in H+ levels, i.e., to acidification. Under the latter conditions, photosynthetic uptake was strongly inhibited. Low-light acclimated cells were able to overcompensate this inhibition in uptake with additional CO2 uptake. High-light acclimated cells were unable to increase CO2 uptake and photosynthesis therefore experienced a shortage in the supply inorganic carbon (Ci). These regulations could be different after an acclimation phase, during which cells adjust their metabolism to the altered conditions, e.g., by changing gene expression. Also, we are currently lacking information about Ci fluxes into calcification and their dependence on carbonation and acidification. In order to understand the differences between short-term and acclimation responses toward carbonation and acidification, we here acclimated E. huxleyi to present-day (ambient [CO2], [ ], and pH) and OA conditions (high [CO2], ambient [ ], low pH). To differentiate between the CO2 and pH/H+ effects, we also acclimated cells to carbonation (high [CO2] and [ ], ambient pH) and acidification (ambient [CO2], low [ ], and pH; Fig. 1; Table 1). We assessed integrated responses in growth, elemental composition and POC and PIC production rates, and measured in vivo fluxes of O2, CO2, and associated with photosynthesis and calcification under acclimation conditions. By comparing these responses with short-term responses (Kottmeier et al. 2016), we aimed to identify processes that were manifested or adjusted over the course of the acclimation. Figure 1Open in figure viewerPowerPoint Decoupled carbonate chemistry during acclimation of Emiliania huxleyi and during cellular flux measurements. Applied conditions were present-day (P; light grey), carbonation (C; dark grey, dashed), acidification (A; white) and ocean acidification (OA; light grey, dashed). Numbers inside the fields denote concentrations of . Concentrations of CO2 and are given in μmol kg−1. Table 1. Carbonate chemistry in the present-day (P), carbonation (C), acidification (A) and the ocean acidification (OA) treatments in cell-free media (Control), at the time of harvesting (Acc), and during cellular flux measurements with membrane-inlet mass spectrometry (MIMS). For acclimation conditions, attained pCO2 (μatm), [H+] (nmol kg−1), DIC (μmol kg−1), [CO2] (μmol kg−1), [ ] (μmol kg−1), [ ] (μmol kg−1), and Ωcalcite were calculated based on measured pHNBS and TA (μmol kg−1) using CO2sys (Pierrot et al. 2006). Results are reported for 15°C (n ≥ 3; ± SD). Input parameters for CO2sys calculations were salinity (31), pressure (0.1 dbar), as well as phosphate (7 μmol kg−1) and silicate (7 μmol kg−1). Equilibrium constants by Mehrbach et al. (1973), refit by Dickson and Millero (1987) and dissociation constants for sulfuric acid by Dickson (1990) were applied. For MIMS conditions, carbonate chemistry was measured mass-spectrometrically (Badger et al. 1994; Schulz et al. 2007). The pCO2 was calculated based on pH and [CO2] after Zeebe and Wolf-Gladrow (2001). Treatment pCO2 pHNBS [H+] TA DIC [CO2] [ ] [ ] Ωcalcite P Control 403 ± 4 8.13 ± 0.00 9.9 ± 0.1 2341 ± 4 2129 ± 4 15 ± 0 1961 ± 5 153 ± 1 3.7 ± 0.0 Acc 384 ± 17 8.14 ± 0.01 9.9 ± 0.1 2280 ± 19 2068 ± 23 15 ± 1 1903 ± 25 151 ± 3 3.7 ± 0.1 MIMS 486 ± 17 8.15 ± 0.01 9.3 ± 0.2 - 2323 ± 180 21 ± 3 2252 ± 264 160 ± 5 - C Control 868 ± 109 8.16 ± 0.02 9.5 ± 0.1 5317 ± 560 4899 ± 529 33 ± 4 4493 ± 489 373 ± 38 9.1 ± 0.9 Acc 805 ± 84 8.18 ± 0.00 8.9 ± 0.3 5223 ± 527 4791 ± 491 31 ± 3 4379 ± 450 382 ± 39 9.3 ± 0.9 MIMS 883 ± 13 8.18 ± 0.02 8.5 ± 0.4 - 4648 ± 69 36 ± 0 4263 ± 64 333 ± 5 - A Control 418 ± 12 7.83 ± 0.00 20.1 ± 0.2 1122 ± 19 1056 ± 19 16 ± 0 1002 ± 19 38 ± 0 0.9 ± 15 Acc 410 ± 51 7.83 ± 0.04 19.0 ± 1.1 1119 ± 31 1052 ± 37 16 ± 2 997 ± 37 39 ± 2 1.0 ± 0.1 MIMS 405 ± 12 7.88 ± 0.02 17.3 ± 0.8 - 1037 ± 31 17 ± 1 980 ± 30 38 ± 1 - OA Control 998 ± 15 7.78 ± 0.01 22.5 ± 0.2 2312 ± 2 2238 ± 2 38 ± 1 2127 ± 2 73 ± 1 1.8 ± 0.0 Acc 964 ± 8 7.79 ± 0.00 22.1 ± 0.2 2287 ± 4 2211 ± 4 37 ± 0 2100 ± 4 73 ± 1 1.8 ± 0.0 MIMS 942 ± 28 7.87 ± 0.02 17.8 ± 0.7 - 2357 ± 70 38 ± 1 2230 ± 67 85 ± 3 - Methods Acclimations Emiliania huxleyi strain RCC1216 was acclimated to four different carbonate chemistry conditions ("present-day", "carbonation", "acidification" and "OA"; Fig. 1; Table 1) under saturating irradiance (400 ± 30 μmol photons m−2 s−1) for 7–14 d, i.e., 10–20 generations. Cells were grown as semicontinuous, dilute batch cultures in a 16 : 8 h light: dark cycle at 15 ± 1°C in sterile-filtered North Sea seawater (0.2 μm, Sartobran 300, Sartorius, Göttingen, Germany) with initial cell concentrations of 1000–3000 cells mL−1 and final concentrations of 40,000–60,000 cells mL−1. Phosphate and nitrate were added to yield concentrations of ∼7 and ∼100 μmol kg−1, respectively. Vitamins and trace metals were adjusted according to F/2 (Guillard and Ryther 1962). Cells were cultured on roller tables in sterilized, gas-tight 2 L borosilicate bottles (Duran Group, Mainz, Germany). Cultures were irradiated by daylight lamps (FQ 54W/965HO, Osram, Munich, Germany). Irradiance was adjusted inside seawater-filled culturing bottles and measured with a Universal Light Meter (ULM 500, Walz, Effeltrich, Germany) using a 4π-sensor (US-SQS/L). Carbonate chemistry was adjusted by aerating the media with humidified, 0.2 μm-filtered air (Midisart 2000, Sartorius) containing a pCO2 of 380 μatm in the present-day and acidification treatments, and a pCO2 of 1000 μatm in the carbonation and OA treatments (Table 1). In the acidification and carbonation treatments, total alkalinity (TA) was adjusted by acid- or base addition (Table 1). Gas mixtures were produced with a gas flow controller (CGM 2000, MCZ Umwelttechnik, Bad Nauheim, Germany), mixing defined portions of pure CO2 (Air Liquide, Duesseldorf, Germany) and CO2-free air (Air purification system, Parker Zander, Kaarst, Germany). Carbonate chemistry of the media was controlled at the beginning as well as at the end of the acclimation period, and was calculated based on pHNBS and TA measurements using CO2sys (Table 1; Pierrot et al. 2006). Shifts in carbonate chemistry over the course of the experiment were small, i.e., drifts in pH were ≤ 0.02 units and TA as well as DIC drifted by ≤ 3%. Measurements of pH were performed with a Metrohm pH meter (826 pH mobile, Metrohm, Filderstadt, Germany) using an Aquatrode Plus electrode with integrated temperature sensor (measurement reproducibility ± 0.01 pH units). TA was determined with potentiometric titration (TitroLine alpha plus, measurement reproducibility ± 7 μmol kg−1, Schott Instruments, Mainz, Germany) of sterile-filtered samples (0.2 μm, cellulose acetate syringe filters, Thermo Fisher Scientific, Waltham, Massachusetts, U.S.A.) and was corrected with certified reference materials (CRM; provided by A. Dickson; Scripps Institution of Oceanography, U.S.A.). Dissolved inorganic carbon (DIC) was controlled with colorimetric measurements of sterile-filtered samples with a QuAAtro autoanalyser (measurement reproducibility ± 5 μmol kg−1, Seal Analytical, Norderstedt, Germany) following the method of (Stoll et al. 2001). Growth and production rates Cellular quotas of POC, PIC, and particulate organic nitrogen (PON; pg cell−1) were measured with an Automated Nitrogen Carbon Analyser mass spectrometer (ANCA-SL 20-20, Sercon Ltd., Crewe, UK). Known volumes of cell suspension were vacuum-filtered (−200 mbar relative to atmosphere) onto precombusted (12 h, 500°C) GF/F filters (1.2 μm; Whatman, Maidstone, UK) 6–8 h after the beginning of the light phase. POC filters were wetted with HCl (200 μL, 0.2 M) to remove calcite and subsequently dried overnight at 65°C prior to measurements. Cellular quotas of PIC were assessed as the difference in carbon quotas between acidified and non-acidified filters. Quotas of chlorophyll a (Chl a; pg cell−1) were assessed by filtering defined volumes of cell suspension onto cellulose nitrate filters (0.45 μm, Sartorius, Göttingen, Germany), which were subsequently frozen in liquid nitrogen and stored at −80°C until analysis. Chl a was extracted in 90% acetone (v/v, Sigma, Munich, Germany) and determined fluorometrically (TD-700 fluorometer, Turner Designs, Sunnyvale, California, U.S.A.) according to Knap et al. (1996). The fluorometer was calibrated with an Anacystis nidulans Chl a standard (Sigma). Cell growth was determined by daily cell counting 6–8 h after the beginning of the light phase with a Coulter Counter (Beckman-Coulter, Fullerton, California, U.S.A.), and the growth constant μ (d−1) was determined as: (1)with c2 and c1 being the cell concentrations (cells mL−1) at the two sampling time points t1 and t2 (d). Production rates of POC and PIC (pg cell−1 d−1) were approximated as (2) (3) Cellular oxygen and carbon fluxes Photosynthetic real time fluxes of oxygen (O2) and Ci were measured by means of membrane-inlet mass spectrometry (MIMS; Isoprime, GV Instruments, Manchester, UK) at conditions resembling the in situ carbonate chemistry (Table 1) and irradiance. Fluxes were estimated following the disequilibrium method by Badger et al. (1994). In this technique, calculations of photosynthetic CO2 and fluxes across the plasmalemma are based on the chemical disequilibrium between the two Ci species during their light-dependent uptake. To account for calcification, we followed the modifications introduced by Schulz et al. (2007) and Kottmeier et al. (2016) and applied measured PIC: POC ratios of the cells that were acclimated to the respective carbonate chemistry conditions. Prior to measurements, acclimated E. huxleyi cells were concentrated by gentle vacuum filtration (−200 mbar relative to atmosphere) over a polycarbonate filter (Isopore TSTP, 3 μm, Merck, Darmstadt, Germany). Culture medium was exchanged with 50 mM N,N-bis(2-hydroxyethyl)glycine (BICINE)-buffered DIC-free seawater medium of the appropriate pH, and 8 mL of the concentrated and buffered cell suspension (5–10 × 106 cells mL−1) were transferred into the MIMS cuvette. Carbonate chemistry was adjusted by adding the corresponding concentrations of NaHCO3 (Table 1). During a first dark phase prior to the actual measurement intervals, membrane-impermeable dextran-bound sulphonamide (25 μM, DBS; Synthelec, Lund, Sweden) was added to inhibit any potential activity of external carbonic anhydrase (CA; please note that this strain expresses hardly any external CA; S. D. Rokitta, unpubl. data). Chl a samples of the concentrated cell suspensions were taken to quantify the assayed biomass. Fluxes of O2 and Ci were measured in consecutive, 6-min light and dark phases in a temperature-controlled cuvette. Steady-state photosynthetic net O2 evolution (Phot; μmol kg−1 min−1) was measured in the light, whereas respiratory O2 uptake (Resp; μmol kg−1 min−1) was measured in the subsequent dark phase. Photosynthetic and respiratory O2 fluxes were converted to Ci fluxes by applying a photosynthetic quotient (PQ) of 1.1 and a respiratory quotient of 1.0, respectively (Burkhardt et al. 2001; Kottmeier et al. 2016). Ci fluxes into calcification (CalMIMS; μmol kg−1 min−1) were derived by multiplying photosynthetic net Ci fixation with light-phase normalized PIC: POC ratios (PIC: POClight) in order to account for continuous respiration of POC during the 8-h dark phase (Schulz et al. 2007): (4) (5) Cellular CO2 uptake (CO2uptot; µmol kg−1 min−1) was deduced from steady-state CO2 drawdown in the light, and corrected for the simultaneous inter-conversion between CO2 and according to Badger et al. (1994). Because calcification is predominantly supplied by external (Sikes et al. 1980; Rost et al. 2002), we assumed that the CO2 uptake for calcification (CO2upCaCO3; µmol kg−1 min−1) was 20% of overall CalMIMS (Kottmeier et al. 2016). Accordingly, uptake for calcification ( upCaCO3; µmol kg−1 min−1) was assumed to be 0.8 × CalMIMS. In order to test how strongly the assumption of 20% CO2 usage for calcification affects the estimated photosynthetic CO2 and fluxes, we performed a sensitivity study, which revealed that errors in this assumption would cause small offsets, but do not change the overall observed regulation patterns (data not shown). Photosynthetic CO2 uptake (CO2upPS; µmol kg−1 min−1) was calculated by subtracting CO2upCaCO3 from overall cellular CO2 uptake. The fraction of overall photosynthetic Ci uptake that is covered by CO2 (fCO2) was obtained according to Kottmeier et al. (2016). Photosynthetic uptake ( upPS; µmol kg−1 min−1) was calculated as the difference between overall photosynthetic Ci uptake and photosynthetic CO2 uptake. Total uptake ( uptot; µmol kg−1 min−1) was calculated as the sum of uptake for calcification and uptake for photosynthesis. All rates were normalized to Chl a. For further details on the calculations of the photosynthetic fluxes, we refer to Kottmeier et al. (2016). Statistics All experiments were carried out in biological triplicates. Differences between the present-day, carbonation, acidification, and OA treatments were tested pairwise for significance by applying two-sided t-tests. Effects were considered statistically significant when p-values were ≤ 0.05. In the Figures and Table 2, significant differences were indicated by different lower-case characters (e.g., a and b). Values denoted by two letters (e.g., ab) represent data that are not significantly different from a and b. Results Integrated responses Cellular growth was unaltered after acclimation to carbonation, but decreased from ∼1.1 at present-day to ∼1.0 d−1 after acclimation to acidification or OA (Fig. 2A; Table 2). Cellular POC production was increased under carbonation (∼15%), but constant under acidification and slightly decreased (∼10%) under OA (Fig. 2B; Table 2). Also PIC production was strongly stimulated under carbonation (∼45%), but decreased under acidification and OA (∼15%; Fig. 2C; Table 2). The ratio of PIC: POC increased by ∼20% under carbonation, but decreased slightly under acidification and OA (Fig. 2D; ∼10%). Cellular Chl a quotas and Chl a: POC ratios, as well as the ratio of POC: PON were not affected by carbonate chemistry (Table 2). Scanning electron microscopy did not reveal malformations of coccoliths under any of the acclimation conditions (data not shown). Figure 2Open in figure viewerPowerPoint Integrated responses to present-day (P; light grey), carbonation (C; dark grey, dashed), acidification (A; white) and ocean acidification (OA; light grey, dashed): (A) Cellular growth constants (μ), (B) production rates of particulate organic carbon (POC) (C) production rates of particulate inorganic carbon (PIC) and (D) PIC: POC ratios. Error bars indicate SD (n = 3). The different lower-case characters indicate significant differences between the data obtained at different carbonate chemistry conditions, e.g., data labeled "a" are statistically different from bars labeled "b" or "c." Table 2. Integrated responses and underlying cellular fluxes of Emiliania huxleyi in the present-day (P), carbonation (C), acidification (A), and ocean acidification (OA) treatments: Different lower-case characters in superscript indicate statistically significant differences between the fluxes obtained at the different the carbonate chemistry conditions. Parameter (unit) P C A OA μ (d−1) 1.16 ± 0.01a 1.15 ± 0.04a 1.02 ± 0.03b 1.01 ± 0.02b POC quota (pg cell−1) 8.9 ± 0.6a 10.2 ± 0.3b 9.7 ± 0.7ab 8.9 ± 0.2a POC production (pg cell−1 d−1) 10.1 ± 0.7a 11.4 ± 0.4b 9.7 ± 0.7a 8.8 ± 0.2c PIC quota (pg cell−1) 10.0 ± 0.3a 14.0 ± 1.0b 9.5 ± 0.5a 9.5 ± 0.3c PIC production (pg cell−1 d−1) 11.3 ± 0.3a 15.7 ± 1.1b 9.5 ± 0.5c 9.2 ± 0.3c PIC: POC (molar ratio) 1.12 ± 0.08a 1.37± 0.08b 0.98 ± 0.03c 1.05 ± 0.03a PIC: POClight (molar ratio) 0.87 ± 0.02a 1.15 ± 0.03b 0.82 ± 0.06a 0.88 ± 0.02a Chl a quota (pg cell−1) 0.13 ± 0.01a 0.13 ± 0.01a 0.12 ± 0.01a 0.12 ± 0.01a Chl a: POC (pg pg−1) 0.014 ± 0.001a 0.013 ± 0.001a 0.013 ± 0.004a 0.014 ± 0.002a PON quota (pg cell−1 d−1) 1.5 ± 0.1a 1.7 ± 0.2a 1.8 ± 0.1a 1.6 ± 0.1a POC: PON (molar ratio) 6.9 ± 0.2a 6.8 ± 0.4a 6.9 ± 0.3a 6.6 ± 0.2a Phot (μmol (mg Chl a)−1 h−1) 284 ± 41a 371 ± 11b 268 ± 41a 295 ± 38a µMIMS (d−1) 1.06 ± 0.15a 1.22 ± 0.04a 0.88 ± 0.13a 1.07 ± 0.12a CO2upPS (μmol (mg Chl a)−1 h−1) 15 ± 11a −30 ± 17b 93 ± 21c 105 ± 20c upPS (μmol (mg Chl a)−1 h−1) 244 ± 34a 368 ± 27b 151 ± 23c 163 ± 53c fCO2 0.06 ± 0.03a −0.09 ± 0.05b 0.38 ± 0.05c 0.40 ± 0.13c CalMIMS 237 ± 34a 390 ± 18b 202 ± 42a 222 ± 32a CO2upCaCO3 (μmol (mg Chl a)−1 h−1) 47 ± 7a 78 ± 4b 40 ± 8a 50 ± 2a upCaCO3 (μmol (mg Chl a)−1 h−1) 189 ± 28a 312 ± 15b 161 ± 34a 178 ± 25a CO2uptot (μmol (mg Chl a)−1 h−1) 57 ± 15a 48 ± 14b 134 ± 29a 156 ± 20b uptot (μmol (mg Chl a)−1 h−1) 448 ± 32a 679 ± 41b 312 ± 54c 352 ± 80c upCaCO3: uptPS 0.77 ± 0.03a 0.85 ± 0.03a 1.07 ± 0.15b 1.22 ± 0.28b Resp (μmol (mg Chl a)−1 h−1) 100 ± 27a 107 ± 12a 77 ± 16a 85 ± 4a Phot: Resp 3.0 ± 0.8a 3.5 ± 0.4a 3.6 ± 1.1a 3.5 ± 0.4a Cellular fluxes We measured cellular O2 and Ci fluxes of the acclimated cells under in situ carbonate chemistry and light conditions in order to identify the alterations in fluxes that caused the alterations in the integrated responses. Similar to the POC production, also Chl a-normalized O2 evolution (Phot) indicated that photosynthesis was increased under carbonation (∼30%), but unaffected by acidification or OA (Fig. 3A; Table 2). Photosynthetic CO2 uptake (CO2upPS) was low under present-day, became negative under carbonation (i.e., cells exhibited a CO2 net efflux), but increased under acidification and OA (∼600%; Fig. 3B; Table 2). Photosynthetic uptake ( upPS) was generally high and was further stimulated by carbonation (∼45%), but decreased under acidification and OA (∼50%; Fig. 3C; Table 2). As a consequence of these antagonistic regulations in CO2 and uptake, the ratio of photosynthetic CO2 uptake to the overall photosynthetic Ci uptake (fCO2) decreased from ∼0.1 to ∼−0.1 under carbonation, but increased to ∼0.4 under acidification and OA (Fig. 3D; Table 2). Respiration (Resp) and the ratio of net photosynthesis to respiration (Phot: Resp) were relatively constant in all applied carbonate chemistry treatments (Table 2). Figure 3Open in figure viewerPowerPoint Cellular O2 and Ci fluxes of Emiliania huxleyi in the present-day (P; light grey), carbonation (C; dark grey, dashed), acidification (A; white), and ocean acidification (OA, light grey, dashed) treatments: (A) photosynthetic net O2 evolution (Phot), (B) photosynthetic CO2 uptake (CO2upPS), (C) photosynthetic uptake ( upPS), (D) ratio of photosynthetic CO2 uptake to overall photosynthetic Ci uptake (fCO2), (E) calcification rates (CalMIMS), (F) uptake for calcification ( upCaCO3), (G) Total uptake ( uptot), (H) Ratio of uptake for calcification to uptake for photosynthesis ( CaCO3: PS). All rates were normalized to Chl a. Error bars indicate SD (n = 3). Different lower-case characters indicate significant differences between the fluxes obtained at different carbonate chemistry conditions. Calcification as estimated from light-normalized PIC: POC ratios and MIMS measurements (CalMIMS) strongly increased under carbonation (∼60%), but apparently stayed constant under acidification and OA (Fig. 3E; Table 2). Yet, CalMIMS seemed to be slightly decreased in both low-pH treatments (Fig. 3E; Table 2). Also CO2 and uptake for calcification (CO2upCaCO3, upCaCO3) increased under carbonation (∼60%), but remained relatively constant under acidification and OA (Fig. 3F; Table 2). Total cellular CO2 uptake (CO2uptot), i.e., the sum of CO2 uptake for photosynthesis and for calcification, was unaffected by carbonation, whereas it strongly increased under acidification and OA (∼150%; Table 2). Total cellular uptake ( uptot) was increased under carbonation (∼50%), whereas it decreased under acidification and OA (∼25%; Fig. 3G; Table 2). The ratio of uptake for calcification to uptake for photosynthesis ( CaCO3: PS) was not affected by carbonation, but strongly increased under acidification and OA (∼50%, Fig. 3H; Table 2). Discussion In this study, we measured the differential effects of acclimation to carbonation and acidification on growth, elemental composition and production rates in high-light grown E. huxleyi. In order to explain the observed integrated cellular responses, we measured the in vivo O2, CO2, and fluxes of the acclimated cells under in situ conditions by means of MIMS. Acclimation to carbonation boosts POC and PIC production by stimulating the uptake of Rates of POC production were strongly increased in the carbonation treatment, but were relatively unaffected under acidified conditions, i.e., in the acidification and OA treatments (Fig. 2B). The increase in biomass buildup under carbonation was also reflected in elevated rates of photosynthetic net O2 evolution (Fig. 3A). Besides POC production, also PIC production and the MIMS-based estimates of calcification were significantly elevated under carbonation (Figs. 2C, 3E, 4B). The carbonation-driven increase in PIC production was larger than the increase in POC production, i.e., PIC: POC ratios increased (Fig. 2D; Table 2). This suggests that, when photosynthesis is substrate-saturated, the residual Ci is directed towards calcification. A redirection of Ci from photosynthesis to calcification was also observed under nutrient limitation, when cells cannot sustain photosynthetic biomass production and excess Ci is therefore available (Paasche and Brubak 1994; Van Bleijswijk et al. 1994; Paasche 1998). On the other hand, PIC: POC ratios were shown to decrease when DIC levels become too low to sustain both processes (Buitenhuis et al. 1999; Zondervan et al. 2002; Bach et al. 2013). Under these conditions, maintaining photosynthesis seems to be more important than sustaining calcification. Apparently, increased calcification acts as a "sink" for excess Ci, while decreased calcification acts as a Ci "source" for photosynthesis when intracellular Ci becomes sparse. Figure 4Open in figure viewerPowerPoint Schematic illustration of Ci fluxes in Emiliania huxleyi acclimated to different carbonate chemistry settings under high irradiances: (A) Under present-day conditions, is the main external substrate for photosynthesis and calcification. Fluxes of into photosynthesis are slightly higher than fluxes
更多查看译文
AI 理解论文
溯源树
样例
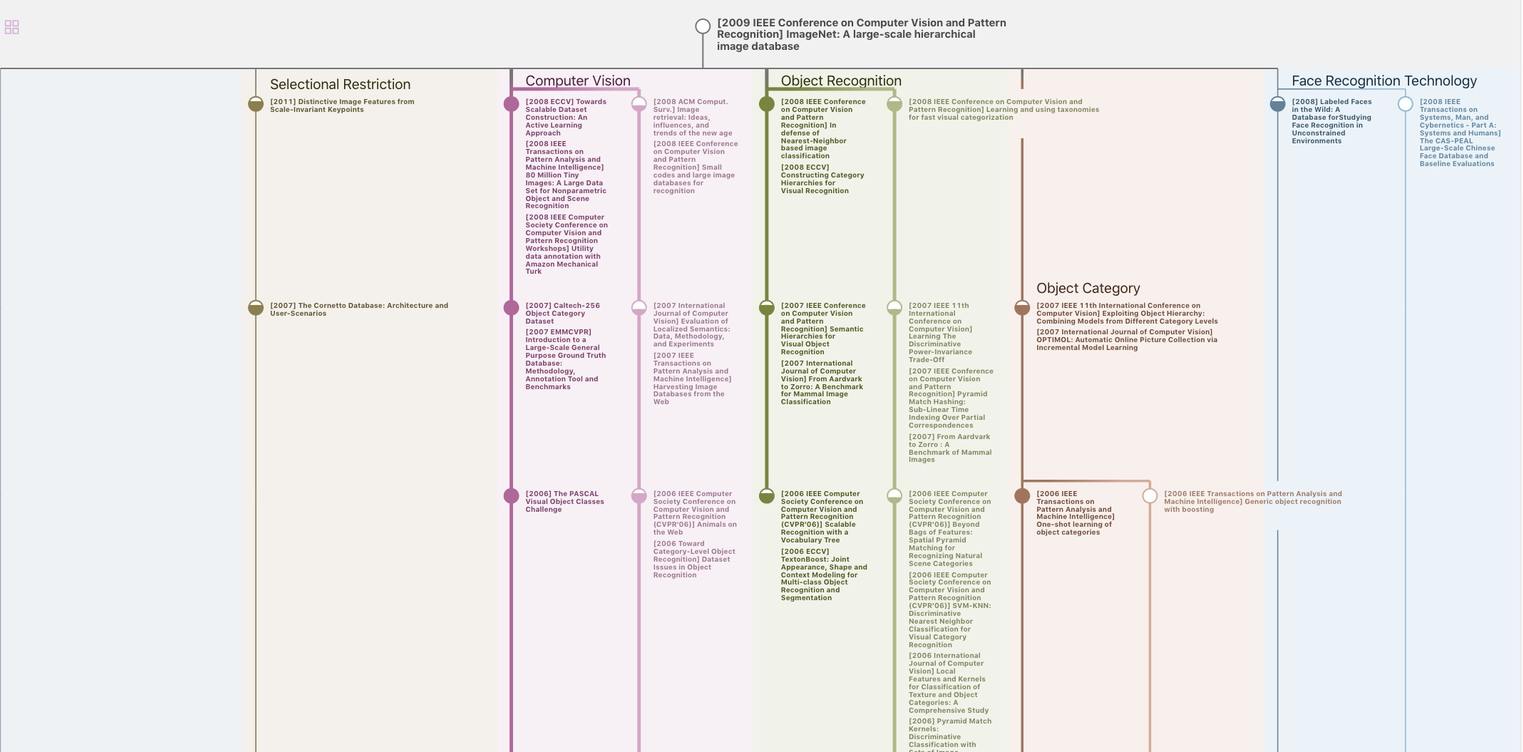
生成溯源树,研究论文发展脉络
Chat Paper
正在生成论文摘要