Room-Temperature Persistent Luminescence in Metal Halide Perovskite Nanocrystals for Solar-Driven CO 2 Bioreduction
CCS Chemistry(2022)
摘要
Open AccessCCS ChemistryRESEARCH ARTICLE11 Mar 2022Room-Temperature Persistent Luminescence in Metal Halide Perovskite Nanocrystals for Solar-Driven CO2 Bioreduction Jie Wang†, Na Chen†, Wenjie Wang, Zhiheng Li, Bolong Huang, Yanbing Yang and Quan Yuan Jie Wang† College of Chemistry and Molecular Sciences, School of Microelectronics, Wuhan University, Wuhan 430072 †J. Wang and N. Chen contributed equally to this work.Google Scholar More articles by this author , Na Chen† College of Chemistry and Molecular Sciences, School of Microelectronics, Wuhan University, Wuhan 430072 †J. Wang and N. Chen contributed equally to this work.Google Scholar More articles by this author , Wenjie Wang Molecular Science and Biomedicine Laboratory (MBL), Institute of Chemical Biology and Nanomedicine, State Key Laboratory of Chemo/Biosensing and Chemometrics, College of Chemistry and Chemical Engineering, Hunan University, Changsha 410082 Google Scholar More articles by this author , Zhiheng Li College of Chemistry and Molecular Sciences, School of Microelectronics, Wuhan University, Wuhan 430072 Google Scholar More articles by this author , Bolong Huang Department of Applied Biology and Chemical Technology, The Hong Kong Polytechnic University, Hung Hom, Kowloon, Hong Kong SAR 999077 Google Scholar More articles by this author , Yanbing Yang *Corresponding authors: E-mail Address: [email protected] E-mail Address: [email protected] College of Chemistry and Molecular Sciences, School of Microelectronics, Wuhan University, Wuhan 430072 Google Scholar More articles by this author and Quan Yuan *Corresponding authors: E-mail Address: [email protected] E-mail Address: [email protected] College of Chemistry and Molecular Sciences, School of Microelectronics, Wuhan University, Wuhan 430072 Molecular Science and Biomedicine Laboratory (MBL), Institute of Chemical Biology and Nanomedicine, State Key Laboratory of Chemo/Biosensing and Chemometrics, College of Chemistry and Chemical Engineering, Hunan University, Changsha 410082 Google Scholar More articles by this author https://doi.org/10.31635/ccschem.022.202101694 SectionsSupplemental MaterialAboutAbstractPDF ToolsAdd to favoritesDownload CitationsTrack Citations ShareFacebookTwitterLinked InEmail The rapid crystal growth of metal halide perovskite (MHP) nanocrystals inevitably leads to the generation of abundant crystal defects in the lattice. Here, defects-mediated long-lived charges and accompanying room-temperature persistent luminescence are demonstrated to be a general phenomenon in MHP nanocrystals. Density functional theory calculations suggest that the collaboration of Schottky and point defects enables upward cascading depletion for electron transfer in MHP nanocrystals, leading to the generation of long-lived photoexcited charges with lifetimes over 30 min. The excellent optical properties including the presence of long-lived charges, high charge separation efficiency, and broad absorption in the visible region make MHPs ideal candidates for both photocatalysis and photobiocatalysis. The MHPs were further integrated with enzymes to construct a light-driven biosynthetic system for the selective production of fine chemicals from CO2 with solar energy. The biosynthetic system can produce formate with a quantum yield of 3.24%, much higher than that of plants (∼0.2–1.6%). These findings will benefit the understanding of the optoelectronic properties of MHPs and further provide opportunities for the development of biosynthetic systems for solar-to-chemical synthesis. Download figure Download PowerPoint Introduction Metal halide perovskites (MHPs) have emerged as a fascinating class of semiconductors with superior optoelectronic properties, including a large light absorption coefficient, high charge mobility, long charge diffusion length, and tunable bandgaps.1–4 In recent years, high-performance optoelectronics, such as solar cells,5–7 light-emitting diodes (LEDs),8 lasers,9 and photodetectors,1,2,10 have been developed based on MHPs.4,11 Within the short time since their development, perovskite solar cells have achieved power conversion efficiencies approaching that of commercialized polycrystalline silicon photovoltaics.1,12 The optoelectronic properties of MHPs are highly correlated with their lattice structures.13–15 Researchers have shown that regulating the lattice structures in MHPs by means such as doping13–15 can change their crystalline structure, electronic structure, bandgap, and crystallinity of MHPs, leading to controllable quantum yields,8 increased charge transport, and stability.14–17 The lattice structure is one of the chief factors that determines the optoelectronic properties of MHPs. Defects, the ubiquitous lattice structures in solid materials, are deviations or disruptions in the continuity of the regular arrangement of atoms.18 The most exciting feature of MHPs is their defect tolerance,1,2,4 indicating the presence of a large number of defects in MHPs.19,20 Generally, the rapid crystal growth of MHPs inevitably leads to the generation of abundant defects in the lattice, such as vacancies and interstitials.1,4,21 Defects in semiconductors have always been of paramount importance as they control the optoelectronic performance of these materials in applications.22,23 The presence of defects can form transition levels in the bandgap and change the absorption/emission properties of semiconductors, leading to a substantial enhancement of the photoluminescence quantum yields or red shift of the emission peaks.23,24 Also, defects can trap free charges and lead to the generation of long-lived charges with lifetimes up to hours.23,25,26 The long-lived charges can recombine to produce persistent luminescence26–28 or be transported to the surface of the semiconductors for photocatalysis.23 The large number of unavoidable defects in MHPs also leads to modification of their bandgaps and the entrapment of free charges; that is, the defects empower MHPs with undiscovered optoelectronic properties. Herein, we report that the intrinsic defects in MHPs can lead to long-lived charge separation and the persistent luminescence phenomenon, and the MHPs were integrated with enzymes for the selective production of fine chemicals from CO2. Persistent luminescence was observed as a general phenomenon in MHPs due to the recombination of the long-lived charges after excitation ceases. The formation of different defects in CsPbBr3 was studied with density functional theory (DFT) calculations, which indicated that the collaboration of Schottky defects and point defects supports the formation of long-lived charges based on the broad distribution of occupied energy levels within the bandgap. A light-driven biosynthetic system was further constructed for the production of fine chemicals from CO2 based on CsPbBr3 and enzymes. This biosynthetic system can produce formate from CO2 continuously over light-dark cycles, demonstrating a promising route toward solar-to-chemical CO2 reduction. Experimental Methods Materials Lead(II) chloride (PbCl2, 99.99%), lead(II) bromide (PbBr2, 99.0%), lead(II) iodide (PbI2, 99.9%), caesium carbonate (Cs2CO3, 99.9%), bismuth triiodide (BiI3, 99.99%), silver nitrate (AgNO3, 99.8%), oleic acid (OA, AR), 1-octadecene (ODE, 80–90%), oleylamine (OLA, AR), hydrobromic acid (HBr, AR, 40%), titanium butoxide (TBOT, ≥99.0%), triethanolamine (TEOA, AR), 2,2′-bipyridyl (AR, 99.0%), pentamethylcyclopentadienylrhodium(III) chloride dimer ((Cp*RhCl2)2, 99%), and β-nicotinamide adenine dinucleotide (NAD+, 97%) were purchased from Aladdin Reagent Co. Ltd. (Shanghai, China). Toluene (High Performance Liquid Chromatography grade) was purchased from Thermo Fisher Scientific, Inc. (Shanghai, China). Bismuth tribromide (BiBr3, 99%) was purchased from Alfa Aesar Chemical Co. Ltd. (Shanghai, China). Methanol (AR, ≥ 99.7%), ethyl acetate (AR, ≥ 99.5%), n-hexane (AR, ≥ 97.0 %), and diethyl ether (AR, ≥ 99.5%) were purchased from Sinopharm Chemical Reagent Co., Ltd. (Shanghai, China). Formate dehydrogenase from Candida boidinii (CbFDH) and formate assay kit were purchased from Sigma-Aldrich Co., LLC. (Shanghai, China). Characterization The sizes and shapes of nanocrystals were determined by a transmission electron microscope (TEM) (JEOL, JEM-2100, Japan). The elemental mappings were conducted on a JEOL JEM-2100F TEM working at 200 kV. The shapes and elemental mappings of p-CsPbBr3 were characterized by a field-emission scanning electron microscope (Zeiss Merlin Compact, Germany). Powder X-ray diffraction (XRD) characterization was conducted on a X-ray diffractometer (Burker, D8 Advance, Germany) with Cu-Kα radiation (λ = 1.5406 Å). Absorption spectra of nanocrystals were collected with a UV–vis absorbance spectrometer (Shimadzu, UV-2550, Japan). Photoluminescence spectra of nanocrystals were measured on a fluorescence spectrometer (Hitachi, FL4600, Japan). The afterglow luminescence in the CsPbBr3 nanocrystal powder was collected on a fluorescence spectrometer (HORIBA Scientific, United States), Fluorolog-3-2ultrafast with 450 W xenon lamp, double mono in ex side, single mono (with H10330 NIR PMT as T-side) and iHR320 (with PPD-850 and CCD as L-side) in Em sides. The photoluminescence decay spectra were recorded on an fluorescence spectrometer (FLS980, Edinburgh Instruments) using the time-correlated single-photon counting mode. X-ray photoelectron spectroscopy (XPS) was analyzed using a X-ray photoelectron spectrometer (Escalab 250Xi, Thermo Fisher Scientific) equipped with Al Kα monochromatized X-rays at 1486.6 eV. The photoluminescence and persistent luminescence photos and videos were taken with a digital single-lens reflex camera (Nikon, D3000, Japan), and a portable ZF5 UV lamp was used as the excitation source. DFT calculations The CASTEP package within DFT was applied to perform the theoretical calculations for CsPbBr3.29 The generalized gradient approximation with Perdue–Burke–Ernzerhof was employed to show the exchange-correlation energy in all of the calculations.30,31 The ultrasoft pseudopotential (cutoff energy, 310 eV) was used. The Broyden–Fletcher–Goldfarb–Shanno (BFGS) algorithm was employed to converge the Hellmann–Feynman forces to <0.001 eV/Å for all geometry optimization.32 Guided by the initial convergence test, Monkhorst–Pack reciprocal space integration was conducted with coarse k-points in consideration of the DFT computational cost.33 The ultrafine convergence criteria were set according to the tolerances of the interionic displacement, and the total energy did not exceed 5 × 10−3 Å per atom and 5 × 10−5 eV per atom, respectively. Measurement of the afterglow decay images A colloidal mixture of nanocrystals in n-hexane was placed in a 48-well plate. A portable ZF5 UV lamp was used as the excitation source. After illumination for 3 min, the decay images were recorded by using the IVIS Lumina XR Imaging System (Caliper, Peoria, IL, United States). Photoelectrochemical measurement The photoelectrochemical properties of p-CsPbBr3 nanocrystals were measured on a CHI Model 618 C electrochemical workstation. A standard three-electrode system was used: fluorine-doped tin oxide glass coated with p-CsPbBr3 nanocrystals as the working electrode, Pt plate as the counter electrode, and Ag/AgCl as the reference electrode. The 0.5 M Na2SO4 electrolyte solution was purged with high-purity argon for 30 min before each measurement. The photocurrent curves were recorded using a 300 W xenon lamp with switch on–off cycles. Synthesis of Cs-oleate A stock solution of Cs-oleate was synthesized following the reported synthesis procedure.34 Briefly, 0.8145 g of Cs2CO3 (2.5 mmol), 2.5 mL of OA, and 40 mL of ODE were added to a 100 mL flask, followed by drying at 120 °C for 1 h under vacuum. After that, the solution in the flask was heated at 150 °C under argon gas. The solution became clear, and the obtained Cs-oleate was collected for further use. Synthesis of CsPbX3 nanocrystals The CsPbX3 perovskite nanocrystals were prepared according to a previously reported hot-injection method.6 Briefly, 10 mL of ODE and 0.38 mmol of PbX2 were added to a 50 mL three-neck round bottom flask, followed by drying for 1 h at 120 °C under vacuum. Then 1 mL of OLA and 1 mL of OA were injected into the above solution at 120 °C under argon gas. After PbX2 was dissolved completely, the solution in the flask was heated at 160 °C. Then 1 mL of Cs-oleate solution preheated at 100 °C was quickly injected into the mixture. After 10 s of reaction, the flask was transferred to an ice-water bath for rapid cooling. The CsPbX3 nanocrystals were collected by centrifugation. Synthesis of Cs2AgBiBr6 nanocrystals The Cs2AgBiBr6 nanocrystals were synthesized according to a reported procedure.35 Briefly, 45 mg of BiBr3, 17 mg of AgNO3, 0.1 mL of HBr, 4 mL of ODE, 1 mL of OA, and 1 mL of OLA were loaded into a 25 mL three-neck round bottom flask and were heated at 120 °C for 1 h under vacuum. After that, the solution was heated at 200 °C in argon gas, and 0.8 mL of preheated Cs-oleate stock solution (100 °C) was quickly added into the solution under vigorous stirring. The color of the mixture quickly turned from yellow-green to yellow. A few seconds later, the flask was rapidly placed in an ice-water bath for cooling. The obtained Cs2AgBiBr6 nanocrystals were collected by centrifugation and redispersed in n-hexane. Synthesis of Cs3Bi2I9 nanocrystals The Cs3Bi2I9 nanocrystals were synthesized by a reported protocol.36 The Cs-oleate precursor and the BiI3 precursor were synthesized first. OA (1.5 mL), ODE (20 mL), and Cs2CO3 (0.45 g) were added to a 50 mL three-neck round bottom flask. The mixture was heated at 130 °C for 1 h with stirring under vacuum to form a clear colloidal Cs-oleate precursor. After that, OA (1.5 mL), OLA (1.5 mL), ODE (15 mL), and BiI3 (330 mg) were added to a 50 mL three-neck round bottom flask. The mixture was dried under vacuum for 1 h at 90 °C to generate a yellow colloidal BiI3 precursor. In a typical procedure, 2 mL of BiI3 precursor in a glass vial was heated at 100 °C for 5 min. Then 0.1 mL of the preheated Cs-oleate solution was quickly added to the above solution. After about 40 s of reaction, the solution changed from yellow to deep orange, indicating the formation of Cs3Bi2I9 nanocrystals. The Cs3Bi2I9 nanocrystals were separated by centrifugation and redispersed in n-hexane. Synthesis of p-CsPbBr3 nanocrystals The p-CsPbBr3 nanocrystals were synthesized following the reported method.37 First, the TBOT solution was prepared by dissolving 20 μL TBOT in 1 mL toluene, and the solution was added dropwise to 10 mL of CsPbBr3 solution (about 2 mg/mL toluene) in a 50 mL centrifuge tube under stirring. The obtained solution was stirred at room temperature for hydrolysis until the clear solution became cloudy. After 3 h of reaction, the precipitate was separated by centrifugation, followed by drying at room temperature for 1 h and 80 °C overnight under vacuum. Subsequently, the dried yellow product was calcined at 300 °C for 5 h under argon flow. Finally, the p-CsPbBr3 nanocrystals were ground in an agate mortar and stored in a centrifuge tube for further use. Preparation of [Cp*Rh(bpy)H2O]2+ Briefly, 30.90 mg of (Cp*RhCl2)2 was suspended in 4 mL of methanol, and a red suspension was formed. Then 15.62 mg of 2,2′-bipyridyl was added to the red suspension, and a clear yellowish solution was obtained after a few minutes. The above yellowish solution was concentrated to about 1 mL by rotary evaporation. After that, [Cp*Rh(bpy)Cl]Cl was collected by adding diethyl ether to the concentrated solution at 4 °C and dried under vacuum conditions. A stock solution of [Cp*Rh(bpy)(H2O)]2+ (50 mM) was prepared by adding [Cp*Rh(bpy)Cl]Cl to 1 mL of water and stored at 4 °C in the dark. Photocatalytic regeneration of NADH The photocatalytic reduction of NAD+ into NADH (NADH = reduced form of nicotinamide-adenine dinucleotide) was conducted in a quartz reactor, and a 450 nm LED (50 W) was used to excite the solution. The photocatalytic reaction system (1 mL) contained 1 mM of NAD+, 0.125 mM of [Cp*Rh(bpy)H2O]2+, 2 M of TEOA, 0.5 mg/mL p-CsPbBr3 and 100 mM phosphate buffer (PB, pH 7.5). The reaction system was incubated for 10 min in darkness before being illuminated by a 450 nm LED. The concentration of the regenerated NADH was determined by UV–vis spectrophotometry using 6220 M−1 cm−1 as the extinction coefficient of NADH at 340 nm. To optimize the catalytic performance of p-CsPbBr3 nanocrystals, the pH values, sacrificial reagents, and the concentration of TEOA and [Cp*Rh(bpy)H2O]2+ were manipulated. Long persistent photocatalytic regeneration of NADH The long persistent photocatalytic production of NADH was conducted with a similar protocol. First, p-CsPbBr3 or TiO2 nanoparticles (2 mg) dispersed in 0.2 mL of PB buffer (100 mM, pH 7.5) was illuminated by a 50 W 450 nm LED lamp for 2 h. Then, the irradiated solution was added to a reaction system composed of 1 mM of NAD+, 0.125 mM of [Cp*Rh(bpy)H2O]2+, 2 M of triethanolamine, and PB buffer. The final volume of the reaction system was 1 mL. The reaction system was incubated in the dark, and the amount of the regenerated NADH was determined by UV–vis spectrophotometry. Biosynthesis of formate from CO2 Briefly, the biosynthesis of the formate was conducted in a glass vial bubbled with CO2 gas at room temperature, and a 50 W 450 nm LED was used as the light source. The reaction solution (2 mL) containing 2 mg/mL p-CsPbBr3 or TiO2 nanoparticles, 5 mM NAD+, 0.125 mM of [Cp*Rh(bpy)H2O]2+, 2 M of triethanolamine, 2 mg/mL CbFDH, and 100 mM PB buffer (pH 7.5) was bubbled with CO2 gas. After illumination with a 450 nm LED for the designated time, the supernatant was collected by centrifugation. The concentration of formate in the supernatant was detected with the formate assay kit. Biosynthesis of formate from CO2 in light-dark cycles The biosynthesis of formate with a light-dark cycle was carried out similarly to the above reaction. For the biosynthesis reaction under light illumination, a reaction system (2 mL) containing 2 mg/mL p-CsPbBr3, 5 mM NAD+, 0.125 mM of [Cp*Rh(bpy)H2O]2+, 2 M of triethanolamine, and 100 mM PB buffer (pH 7.5) was loaded into a glass vial and illuminated with 450 nm LED lamp for a certain time. For the dark stage, 4 mg of CbFDH was added to the obtained solution, and the solution was bubbled with CO2 gas in the dark at room temperature. The formate concentration was monitored by a formate assay kit. The artificial photosynthesis of the formate with four light-dark cycles was performed in a glass vial bubbled with CO2 gas under the same reaction conditions. Average photoluminescence lifetime calculation The transient photoluminescence decay curves of CsPbBr3 and p-CsPbBr3 nanocrystals were fitted with a triplet-exponential function of Y(t) = A1exp(−t/τ1) + A2exp(−t/τ2) + A3exp(−t/τ3) + y0, and the average photoluminescence lifetime (τ) of CsPbX3 and p-CsPbBr3 nanocrystals were calculated from eq 1: τ = A 1 τ 1 2 + A 2 τ 2 2 + A 3 τ 3 2 A 1 τ 1 + A 2 τ 2 + A 3 τ 3 . (1) Quantum yield calculation According to a previous study, the formate production quantum yield was calculated by the ratio of the effective electrons used for formate production to the total input photon flux38: Quantum yield ( % ) = 2 × mol of formate mol of total photons × 100 % , (2)where mol of formate generated = the total formate concentration × total volume; mol of total photons = photo flux × area of illumination × reaction time/Avogadro’s number (NA). And photo flux was estimated by eq 3: Photo flux = P × λ h × c , (3)where P is the power of the LED light, λ is the emission wavelength of the LED light, h is the Planck constant (6.63 × 10–34 J·s), and c is the speed of light (3.00 × 108 m·s–1). Results and Discussion The general persistent luminescence phenomenon in MHP nanocrystals The defect-tolerance virtue of MHPs suggests the presence of abundant defects in them.1,2,4 Considering the unique charge-trapping feature of crystal defects, we reason that the long-lived charges and the accompanying persistent luminescence occur in MHPs, as illustrated in Figure 1a. The classic cubic CsPbBr3 nanocrystals34 (Figure 1b) were first tested. As shown in Figure 1c, green persistent luminescence was observed from the CsPbBr3 nanocrystal powder at room temperature after removing the 365 nm UV illumination ( Supporting Information Video S1). Figure 1d further shows that obvious persistent luminescence at around 536 nm was detected in CsPbBr3 nanocrystals even at a 5 s delay after photoexcitation at room temperature. We further recorded the persistent luminescence decay in CsPbBr3 nanocrystals over time. Persistent luminescence with persistent time over 300 s was detected in CsPbBr3 nanocrystals (Figure 1e), suggesting the presence of long-lived charges in CsPbBr3. These results confirm that long-lived charges and the accompanying persistent luminescence occurred in the CsPbBr3 nanocrystals. Figure 1 | Persistent luminescence in MHP nanocrystals. (a) Schematic representation for the persistent luminescence in MHP nanocrystals. (b) TEM image of the CsPbBr3 nanocrystals. (c) Photographs of CsPbBr3 nanocrystal powder during and after excitation. (d) Room-temperature persistent luminescence spectrum of the CsPbBr3 nanocrystals with a delay time of 5 s. (e) Persistent luminescence decay curve of the CsPbBr3 nanocrystals pre-excited by a UV lamp for 2 min. (f) Room-temperature phosphorescence spectra of the MHP nanocrystals with varying halide compositions. (g) Persistent luminescence decay images in MHP nanocrystals. (h) Photographs of CsPbCl1.5Br1.5, CsPbBr3, and CsPbBr1.5I1.5 colloidal dispersions during and after excitation. Download figure Download PowerPoint We next asked whether persistent luminescence is a general phenomenon in MHP nanocrystals. A series of perovskite nanocrystals were further tested, including CsPbX3 (X= Cl–I),34 Cs2AgBiBr6,35 and Cs3Bi2I936 ( Supporting Information Figures S1–S8). The persistent luminescence feature of these perovskite nanocrystals was tested. Figure 1f presents the room-temperature phosphorescence spectra of the MHP nanocrystals. Long-lifetime emissions were observed over the entire visible spectral region from the MHP nanocrystal dispersions, indicating the persistent luminescence feature of these nanocrystals. The persistent luminescence in the MHP nanocrystals was further studied using the IVIS Spectrum imaging system39 in the bioluminescence mode. After precharging with a 365 nm UV lamp for 2 min, all of the MHP nanocrystals displayed strong persistent luminescence after excitation ceases (Figure 1g and Supporting Information Figure S9). The decay images in Figure 1g show that the persistent luminescence intensities of the MHP nanocrystals decreased slowly and that obvious persistent luminescence was still detected after 1 h of decay. Photographs of CsPbX3 colloidal dispersions during and after excitation at room temperature show obvious persistent luminescence (Figure 1h and Supporting Information Video S2). These assays demonstrate that the long-lived charges and the accompanying persistent luminescence are a general phenomenon in MHPs. The origin of the persistent luminescence phenomenon in MHP nanocrystals The persistent luminescence originates from the slow escape and recombination of the charges trapped by defects in MHP nanocrystals after excitation ceases. The formation of defects in CsPbBr3 and their influences on charge separation were further systematically studied via their electronic structures. In CsPbBr3 crystal, both the bonding and antibonding orbitals near the Fermi level (EF) are predominantly contributed to by Pb (Figure 2a). The Br sites also display the electron-rich feature while Cs atoms show a limited contribution to the electronic distribution (Figure 2a). From the projected density of states, the conduction band minimum (CBM) and valence band maximum (VBM) are both mainly composed of the p-orbitals from Pb-6p and Br-4p (Figure 2b). Previous studies have demonstrated the presence of both defects-related shallow and deep trap states in MHPs.19,20 The formation of commonly encountered native defects such as vacancies and their influence on the optical properties of CsPbBr3 was systematically studied with DFT calculations. For the formation of point defects in CsPbBr3 crystals, the pure vacancies show slightly higher energy costs while the antisite and Frenkel defects were thermodynamically favorable, indicating the presence of unavoidable defects in CsPbBr3 crystals during synthesis (Figure 2c). The single-particle levels of intrinsic defects in CsPbBr3 with different charge states are further summarized. Figure 2d indicates that the point defects are responsible for the formation of the hole traps near the VBM. For the Pb vacancy (VPb), the occupied states were noted in both V Pb 0 and V Pb 2 − , which are 0.31 and 0.33 eV above the VBM, respectively. Meanwhile, an empty state at 0.27 eV above the VBM was identified in the neutral V Pb − , which can alleviate the energy barrier for electron transfer from the conduction band. The additional gap states for V Cs 0 and V Cs − were noticed at 0.53 and 0.31 eV above the VBM, respectively. In particular, we noticed the ladder-like distributions of occupied states from Pb i 0 to Pb i 2 + , which varies from the range of 0.5–2.16 eV above the VBM. These states have supplied the nonequilibrium intrinsic charge states to prolong the luminescence performance after excitation. Moreover, large amounts of occupied states have been formed within the range of 0.6 eV by both the anion and cation Frenkel defects. Therefore, we propose the upward cascading depletion mechanism facilitated by high energy levels of defects from the synthesis. Under excitation, electrons trapped in these states can tunnel through the conduction band to realize deexcitation and accompanying persistent luminescence. Figure 2 | The origin of persistent luminescence in CsPbBr3. (a) The bonding and antibonding orbitals of CsPbBr3. Purple balls, Cs; dark grey balls, Pb; brown balls, Br; blue isosurface, bonding orbitals; green isosurface, antibonding orbitals. (b) The projected density of states of CsPbBr3. (c) Formation energies of point defect in CsPbBr3. (d) The summarized single-particle levels of simple point defects of CsPbBr3 in both charged and neutral states (empty states = red; occupied states = black). (e) The summarized single-particle levels of Schottky and Schottky-like defects of CsPbBr3 in agglomerated and separation types. STK-1, VPbBr2-1, and VCsBr-1 are the agglomerated type, and STK-2, VPbBr2-2, and VCsBr-2 are the separated type of defects. Download figure Download PowerPoint Besides the simple point defects, Schottky and Schottky-like defects were further investigated in agglomerated and separated types (Figure 2e). Both the Schottky and the Schottky-like defects such as PbBr vacancy (VPbBr2) introduce empty gap states above the VBM. For instance, for the agglomerated STK defects (STK-1), two additional electron traps were formed at the CBM, which located at 2.49 and 2.89 eV above VBM, respectively. These two electron traps correspond to photon emission of 498 (violet) and 429 nm (blue), which were close to the experimental results. Similar to the agglomerated STK, the separated STK defect also demonstrated two-gap states at 2.50 and 2.07 eV above VBM, supporting the photoemission of 496 (blue) and 598 nm (orange), respectively. These additional empty states not only act as electron traps but also promote upward cascading depletion-based electron transfer by supplying the appropriate energy levels over the CBM. Therefore, combined with the upward cascading depletion by point defects, the intrinsic defects can lead to the generation of long-lived charges and persistent luminescence in CsPbBr3 crystals. In addtion to the intrinsic defects, introducing exogenous defects by means such as heterovalence doping during preparation can further enhance the intensity and prolong the decay time of persistent luminescence in MHPs.25,27 Light-driven biosynthesis of formate from CO2 based on MHPs Th
更多查看译文
关键词
metal halide perovskite nanocrystals,room-temperature,solar-driven
AI 理解论文
溯源树
样例
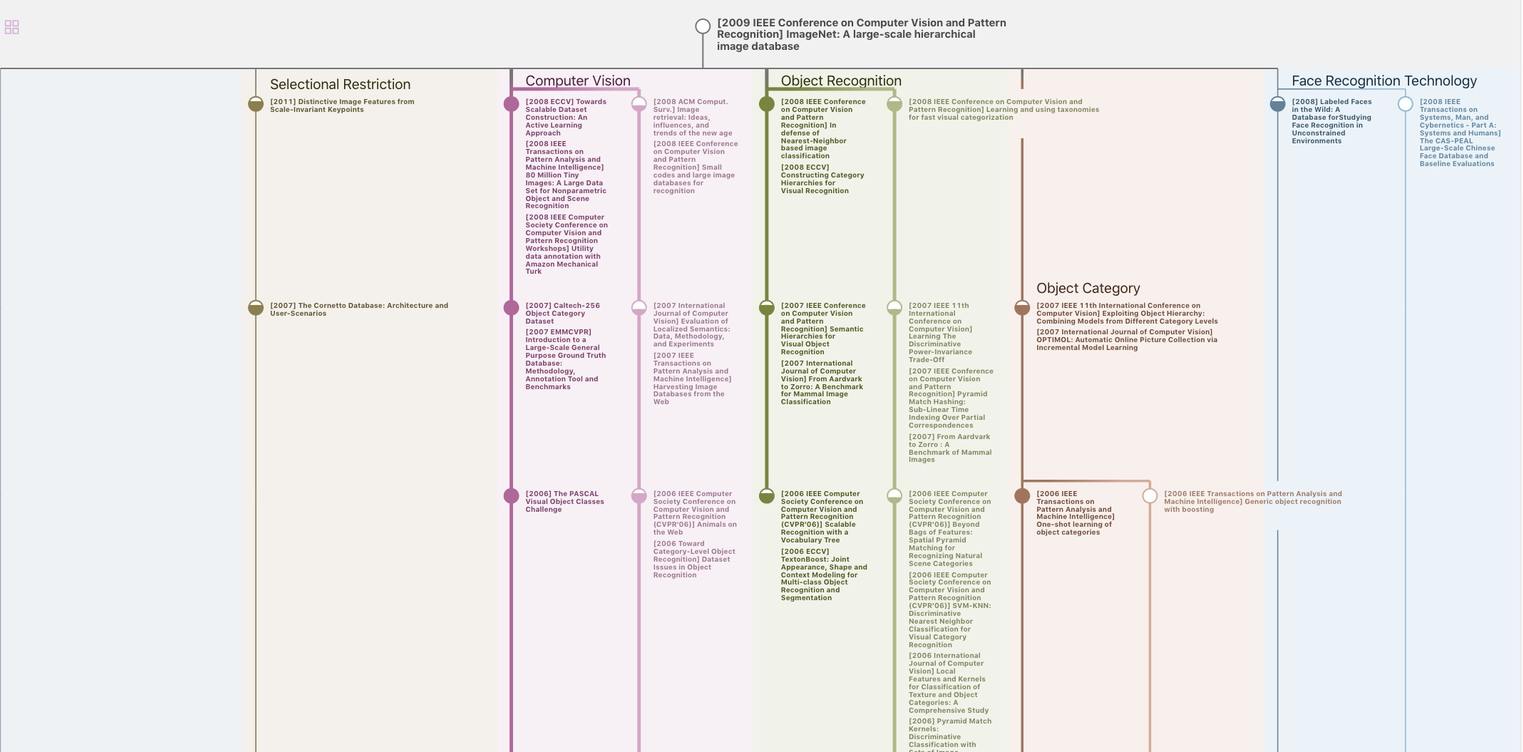
生成溯源树,研究论文发展脉络
Chat Paper
正在生成论文摘要