Fluorous Metal–Organic Frameworks with Unique Cage-in-Cage Structures Featuring Fluorophilic Pore Surfaces for Efficient C 2 H 2 /CO 2 Separation
CCS Chemistry(2022)
摘要
Open AccessCCS ChemistryRESEARCH ARTICLE3 Oct 2022Fluorous Metal–Organic Frameworks with Unique Cage-in-Cage Structures Featuring Fluorophilic Pore Surfaces for Efficient C2H2/CO2 Separation Xing-Ping Fu†, Yu-Ling Wang†, Xue-Feng Zhang, Zhenjie Zhang, Chun-Ting He and Qing-Yan Liu Xing-Ping Fu† College of Chemistry and Chemical Engineering, Key Laboratory of Functional Small Molecules for Ministry of Education, Jiangxi Normal University, Nanchang, Jiangxi 330022 Department of Ecological and Resources Engineering, Fujian Key Laboratory of Eco-Industrial Green Technology, Wuyi University, Wuyishan, Fujian 354300 †X.-P. Fu and Y.-L. Wang contributed equally to this work.Google Scholar More articles by this author , Yu-Ling Wang† College of Chemistry and Chemical Engineering, Key Laboratory of Functional Small Molecules for Ministry of Education, Jiangxi Normal University, Nanchang, Jiangxi 330022 †X.-P. Fu and Y.-L. Wang contributed equally to this work.Google Scholar More articles by this author , Xue-Feng Zhang College of Chemistry and Chemical Engineering, Key Laboratory of Functional Small Molecules for Ministry of Education, Jiangxi Normal University, Nanchang, Jiangxi 330022 Google Scholar More articles by this author , Zhenjie Zhang College of Chemistry and State Key Laboratory of Medicinal Chemical Biology, Nankai University, Tianjin 300071 Google Scholar More articles by this author , Chun-Ting He College of Chemistry and Chemical Engineering, Key Laboratory of Functional Small Molecules for Ministry of Education, Jiangxi Normal University, Nanchang, Jiangxi 330022 Google Scholar More articles by this author and Qing-Yan Liu *Corresponding author: E-mail Address: [email protected] College of Chemistry and Chemical Engineering, Key Laboratory of Functional Small Molecules for Ministry of Education, Jiangxi Normal University, Nanchang, Jiangxi 330022 Google Scholar More articles by this author https://doi.org/10.31635/ccschem.021.202101575 SectionsSupplemental MaterialAboutAbstractPDF ToolsAdd to favoritesDownload CitationsTrack Citations ShareFacebookTwitterLinked InEmail The similarities in molecular size and physical properties of acetylene (C2H2) and carbon dioxide (CO2) produce a formidable challenge for their separation. Herein, we report two isoreticular fluorinated metal–organic frameworks (MOFs), labeled as JXNU-11(Fe2M) (M = Ni and Co), featuring unique octahedral cages encapsulated by cuboctahedral cages. JXNU-11(Fe2Ni) shows a record high C2H2-capture amount of 4.8 mmol g−1 and a long C2H2/CO2 breakthrough interval time of 55 min g−1 in an actual breakthrough experiment based on the equimolar C2H2/CO2 mixture under ambient conditions, indicating a high-performance material for C2H2 capture and C2H2/CO2 separation. Computational simulations revealed that the nanosized double-shell cages decorated with abundant fluorine and carboxylate oxygen atoms afford the optimized pore spaces for preferentially trapping C2H2, which account for the remarkable C2H2-capture capacity and the highly efficient C2H2/CO2 separation for JXNU-11(Fe2M). This work not only develops a design strategy for building the cage-in-cage structures in MOFs, but also provides universal guidance on designing porous MOFs with highly polarized and fluorophilic pore cages to capture C2H2. Download figure Download PowerPoint Introduction Acetylene (C2H2), the simplest alkyne, is an essential chemical for many common chemical products, such as acetaldehyde, acetic acid, plastic, and synthetic rubber,1 and electronic materials in the electronics industry.2 Additionally, C2H2 is an important flammable gas widely utilized for welding and metal cutting. The production of C2H2 from the oxidative coupling of natural gas is a commonly used preparation method for C2H2 gas in the petrochemical industry.3 Such a C2H2 preparation process leads to a small amount of carbon dioxide (CO2) in the C2H2 product. The presence of CO2 in C2H2 starting material has a seriously negative effect on subsequent preparation processes for the down-stream chemical products. Thus separation of C2H2/CO2 mixtures is vital in the petrochemical industry. However, the similar molecular sizes, as well as the same kinetic diameter (3.3 Å) of the linear-shaped C2H2 and CO2 molecules4–9 ( Supporting Information Table S1), cause the C2H2/CO2 separation to be a formidable task. Organic solvent extraction and cryogenic distillation are the current technologies for purifying C2H2 gas but are energy-intensive and environmentally unfriendly. Therefore, it is important to develop a highly energy-efficient and environment-friendly technology for achieving C2H2/CO2 separation. Metal–organic frameworks (MOFs) with porous and crystalline structures are fascinating porous solid materials.5–14 MOFs show highly promising potential in gaseous mixture separation due to their designable frameworks’ structures and tunable pore environments.15–19 Recently, many MOFs have been utilized for the separation of C2H2/CO2 mixtures,20–29 and a few of them exhibit high-performance C2H2/CO2 separation.30–34 In the column breakthrough separation of binary gases, the breakthrough time and the captured amount for the late eluted adsorbate in the column are the important parameters for evaluating the separation efficiency of an adsorbent. The long breakthrough time for the late eluted gas generally results in a large captured amount for the late eluted gas, leading to a high separation efficiency of the adsorbent. However, in practice breakthrough experiments based on the equimolar C2H2/CO2 mixture, C2H2 breakthrough time for MOFs is generally shorter than 50 min g−1 under ambient conditions.35–40 The top-performing MOFs are SNNU-45 and ZJU-74a with the C2H2 breakthrough times of 113 and 81 min g−1,41,42 respectively. In contrast, the C2H2-captured amounts for MOFs in the packed columns are commonly less than 3 mmol g−1. SNNU-45 and ZJU-74a have the highest C2H2-capture amounts of 3.5 and 3.64 mmol g−1, respectively. It has been well demonstrated that the fluorine atoms of the inorganic anions, including MF62− (M = Si4+ and Ti4+) and NbOF52,− in the hybrid MOFs are the preferential bind sites for the acidic hydrogen atoms of the alkyne.43–47 The multiple C–H···F interactions between the C2H2 molecule and inorganic MF62− or NbOF52− groups of MOFs have resulted in an efficient C2H2/CO2 separation.47–49 Thus the fluorinated MOFs are a highly appealing platform for C2H2/CO2 separation. Unfortunately, due to the synthetic inaccessibility of the suitable fluorinated organic ligands, the fluorinated MOFs have thus been largely unexplored.50–54 Herein, we present two isostructural fluorous MOFs [termed as JXNU-11(Fe2M), M represents the divalent metal ions of Ni2+ and Co2+] with a fluorinated organic linker, featuring unique cage-in-cage structures. Remarkably, JXNU-11(Fe2Ni) shows a record-high C2H2-capture amount and a long C2H2 breakthrough time in the practice breakthrough experiment, resulting in high-performance C2H2/CO2 separation. The double-shell cages possessing highly electronegative F and O atoms in JXNU-11(Fe2M) are suitable pore cages to match the size of C2H2. The C2H2 molecules are trapped in the highly fluorophilic and polarized double-shell cages through strong host–guest interactions, which are responsible for the highly efficient C2H2/CO2 separation by JXNU-11(Fe2M). Experimental Section Synthesis of JXNU-11(Fe2Ni) A mixture of FeCl3·6H2O (8.10 mg, 0.03 mmol), Ni(NO3)3·6H2O (4.36 mg, 0.015 mmol), 3,3′,5,5′-tetrakis(fluoro)biphenyl-4,4′-dicarboxylate acid (4.71 mg, 0.015 mmol), 1,3,5-tris(4-carboxyphenyl)benzene (6.58 mg, 0.015 mmol), N,N-dimethylformamide (DMF) (2 mL), and CH3COOH (0.19 mL) was capped in a 20 mL vial and heated at 100 °C for 2 days. After cooling to room temperature, brown triangle-shaped crystals were obtained. Elemental analysis for {[Fe2Ni(μ3-O)(TFBPDC)(BTB)4/3(H2O)3]·6DMF·2.5H2O}n (C68H77F4O24.5N6Fe2Ni: 1616.74). Calcd/found: H, 4.80/4.71; C, 50.51/50.41; N, 5.19/5.02. IR data (KBr, cm−1): 3427 (m), 1662 (s), 1615 (s), 1394 (s), 1253 (w), 1185 (w), 1147 (w), 1098 (w), 1035 (s), 858 (s), 807 (w), 781 (s), 683 (w), 663 (w), 589 (m), 498 (m), 443 (s). Synthesis of JXNU-11(Fe2Co) A mixture of FeCl3·6H2O (8.10 mg, 0.03 mmol), Co(NO3)3·6H2O (4.37 mg, 0.015 mmol), 3,3′,5,5′-tetrakis(fluoro)biphenyl-4,4′-dicarboxylate acid (4.71 mg, 0.015 mmol), 1,3,5-tris(4-carboxyphenyl)benzene (6.58 mg, 0.015 mmol), DMF (2 mL), and CH3COOH (0.17 mL) was capped in a 20 mL vial and heated at 100 °C for 2 days. After cooling to room temperature, brown triangle-shaped crystals were obtained. Elemental analysis for {[Fe2Co(μ3-O)(TFBPDC)(BTB)4/3(H2O)3]·4DMF·H2O}n (C62H60F4O21N4Fe2Co: 1443.77). Calcd/found: H, 4.18/4.21; C, 51.57/51.46; N, 3.88/3.92. IR data (KBr, cm−1): 3420 (m), 1655 (s), 1612 (s), 1394 (s), 1254 (w), 1185 (w), 1146 (w), 1098 (w), 1034 (s), 859 (s), 808 (w), 781 (s), 706 (w), 663 (w), 589 (m), 500 (m), 443 (s). Methods X-ray single-crystal diffraction experiments were carried out with a Rigaku Oxford SuperNova diffractometer, and powder X-ray diffraction patterns were recorded on a Rigaku DMax 2500 powder diffractometer. Gas sorption–desorption isotherms were measured on a Micromeritics ASAP 2020 HD88 adsorption analyzer. Breakthrough experiments for separation of C2H2/CO2 (v/v, 50/50) were carried out in a fixed bed with a gas chromatograph detection system. The detailed experimental methods are provided in Supporting Information. Results and Discussion Compounds JXNU-11(Fe2M) constructed from the oxygen-centered heterometallic trimeric [Fe2M(μ3-O)(COO)6] (M = Ni2+ and Co2+) clusters (Figure 1a) were prepared. Compound JXNU-11(Fe2M) formulated as [Fe2M(μ3-O)(TFBPDC)(BTB)4/3(H2O)3]n is based on the linear 3,3′,5,5′-tetrakis(fluoro)biphenyl-4,4′-dicarboxylate (TFBPDC2−) and the triangular 1,3,5-tris(4-carboxyphenyl)benzene (BTB3−) ligands (Figure 1b) and was characterized by single-crystal X-ray diffraction ( Supporting Information Table S2). The phase purity of the bulk samples was confirmed by powder X-ray diffraction ( Supporting Information Figure S2). The metal concentrations in both compounds were determined by inductively coupled plasma atomic emission spectroscopy ( Supporting Information Table S3). The two compounds are isostructural and crystallize in a trigonal R3m space group. The trimeric [Fe2M(μ3-O)] core is coordinated by two TFBPDC2−, four BTB3−, and three water ligands ( Supporting Information Figure S1). Each [Fe2M(μ3-O)(COO)6] cluster is linked by four BTB3− ligands and two TFBPDC2− ligands to generate a three-dimensional (3D) framework. Exploration of the structure indicates the 3D framework contains two kinds of cages: a small octahedral cage formed from six [Fe2M(μ3-O)(COO)6] clusters, three TFBPDC2−, and four BTB3− ligands with a diameter of ∼12 Å (Figure 1c), and a large cage composed of 12 [Fe2M(μ3-O)(COO)6] clusters, six TFBPDC2−, and ten BTB3− ligands with a diameter of ∼26 Å, which can be described as a cuboctahedron (Figure 1d). Thus the 3D framework has close packing of the octahedral and cuboctahedral cages (Figure 1e). Such large voids in the cages in a single 3D framework lead to the generation of a twofold interpenetrated network (Figure 1f). It is remarkable that each octahedral cage is encapsulated by a large cuboctahedral cage from the interpenetrating network (Figure 1g). Such a unique cage-in-cage structure in a MOF is distinctive. The double-shell nested cages are reminiscent of the interesting Russian-doll-like cage. In addition, because of the presence of the highly polar C–F and C–O bonds and dense F atoms on the pore surfaces for these cages, double-shell nested cages exhibit highly polarized and fluorophilic character. As shown in Figure 1g, part of the voids in the large cuboctahedral cage are occupied by the small octahedral cage. The remaining pores of the total 3D framework are filled by the disordered guest solvent molecules, which occupy 64.1% and 65.6% of the volumes of unit cell for JXNU-11(Fe2Ni) and JXNU-11(Fe2Co), respectively. JXNU-11(Fe2M) materials exhibit excellent chemical stability after exposure to air for a long time or immersion in water with pH values ranging from 3 to 11 for 24 h ( Supporting Information Figure S3). The solvent-free JXNU-11(Fe2M) was obtained through solvent-exchange and heat under vacuum ( Supporting Information Figure S4). Figure 1 | Structures of JXNU-11(Fe2M). (a) [Fe2M(μ3-O)(COO)6] unit and (b) TFBPDC2− and BTB3− ligands. (c) 3D framework of JXNU-11(Fe2M). (d) Octahedral and (e) cuboctahedral cages. (f) Twofold interpenetrating frameworks of JXNU-11(Fe2M). (g) Cage-in-cage structure in JXNU-11(Fe2M). The fluorine atoms in (b–d) are represented as green balls. Download figure Download PowerPoint The permanent porosity of JXNU-11(Fe2M) was confirmed by N2 adsorption isotherms at 77 K. The N2 sorption isotherms for both compounds have typical type I sorption behavior with the saturation sorption amounts of 561 and 556 cm3 g−1 (Figure 2a). The pore volumes for JXNU-11(Fe2Ni) and JXNU-11(Fe2Co) from the experimental N2 data are 0.87 and 0.86 cm3 g−1, respectively, which are close to the corresponding crystal structure calculated values of 0.87 and 0.89 cm3 g−1. Additionally, the pore sizes are mainly around 6.8 and 11 Å (Figure 2a, inset), in good agreement with the obtained values from the crystal structures. The Brunauer–Emmett–Teller surface areas for JXNU-11(Fe2Ni) and JXNU-11(Fe2Co) were calculated to be 2208 m2 g−1 (Langmuir surface area of 2418 m2 g−1) and 2122 m2 g−1 (Langmuir surface area of 2392 m2 g−1) ( Supporting Information Figure S5), respectively. Figure 2 | Adsorption data of JXNU-11(Fe2M). (a) N2 adsorption–desorption isotherms at 77 K and pore size distribution for JXNU-11(Fe2M). C2H2 and CO2 adsorption–desorption isotherms of JXNU-11(Fe2M) at 273 K (b) and 298 K (c). Download figure Download PowerPoint The C2H2 and CO2 sorption isotherms of JXNU-11(Fe2M) (Figures 2b and 2c) were collected to evaluate the gas separation potentials for JXNU-11(Fe2M). JXNU-11(Fe2Ni) and JXNU-11(Fe2Co) exhibit high C2H2 adsorption capacities of 191 and 180 cm3 g−1 at 273 K and 1 bar, respectively, which are higher than the best-performance MOFs, including FJU-6-TATB (160 cm3 g−1),55 ATU-Cu (134 cm3 g−1),33 ZJU-74a (107 cm3 g−1),42 JXNU-10(Y) (94.9 cm3 g−1),27 CuI@UIO-66-(COOH)2 (71 cm3 g−1),56 and MOF-OH (68.7 cm3 g−1).57 At 298 K, the adsorption amounts of C2H2 are 118 and 107 cm3 g−1 for JXNU-11(Fe2Ni) and JXNU-11(Fe2Co), respectively, which are larger than those of the prominent MOFs FeNi-M′MOF (96 cm3 g−1),58 JNU-1 (63 cm3 g−1),59 NKMOF-1-Ni (61 cm3 g−1),60 UTSA-300a (69 cm3 g−1),47 and CPL-NH2 (41 cm3 g−1).39 In contrast, JXNU-11(Fe2M) showed low CO2 uptakes (Figures 2b and 2c). The high uptake of C2H2 is mainly caused by the polarized and fluorophilic microporous environments made of the cage-in-cage structures that are decorated with plenty of highly electronegative fluorine and carboxylate oxygen atoms that have high affinity for the acidic H atoms of C2H2 molecules but low affinity for CO2 molecules with two terminal electronegative O atoms. Such distinct gas sorption behaviors were confirmed by grand canonical Monte Carlo (GCMC) simulations. As depicted in Figures 3a and 3b, the distribution of C2H2 molecules in JXNU-11(Fe2M) is mainly within the octahedral cage and the space between the two cages, whereas much fewer CO2 molecules were distributed within the double-shell nest. Such a phenomenon suggests the double-shell nest is the desirable space for accommodating C2H2 molecules, in agreement with the experimental finding. The linear adsorption isotherms for CO2 further suggest the low affinity to CO2 for the host frameworks. The different adsorption behaviors between C2H2 and CO2 were also evidenced by the isosteric heats of adsorption (Qst) ( Supporting Information Figure S6). The obtained Qst of C2H2 were in the range of 31.6–29.7 kJ mol−1 for JXNU-11(Fe2M), which are notably higher than those of CO2 (16.0–19.6 kJ mol−1), further reflecting the strong affinity toward C2H2. The present Qst(C2H2) values are comparable to those of FJU-6-TATB (29 kJ mol−1),55 UTSA-74 (31 kJ mol−1),37 and JXNU-5a (32.9 kJ mol−1),35 but lower than those of CuI@UIO-66-(COOH)2 (74.5 kJ mol−1),56 JNU-1 (47.6 kJ mol−1),59 and NKMOF-1-Ni (53.9 kJ mol−1).60 The evident difference in adsorption enthalpy endows JXNU-11(Fe2M) with the thermodynamic separation possibility of a C2H2/CO2 mixture. Furthermore, JXNU-11(Fe2M) retained C2H2 storage ability after adsorption/desorption cycling experiments ( Supporting Information Figure S7), confirming their excellent recyclability for C2H2 adsorption. Figure 3 | Grand canonical Monte Carlo (GCMC) adsorption simulation. Computational C2H2 (a) and CO2 (b) distribution in the double-shell nest in JXNU-11(Fe2M) at 298 K and 1 atm. Download figure Download PowerPoint To evaluate the separation performance of JXNU-11(Fe2M), ideal adsorbed solution theory was used to calculate the separation selectivity of the C2H2/CO2 (v∶v = 50∶50) mixture. At 1 bar and 298 K, the adsorption selectivities for JXNU-11(Fe2Ni) and JXNU-11(Fe2Co) are 2.7 and 2.5, respectively ( Supporting Information Figure S8), which are comparable to the leading MOFs of UPC-200(Fe)-F-H2O (2.25)32 and FJU-6-TATB (3.1).55 The C2H2/CO2 uptake ratios for JXNU-11(Fe2Ni) and JXNU-11(Fe2Co) at 298 K and 1 bar are 2.1 and 1.9, respectively, which are larger than those of noted MOFs FJU-90a (1.75),36 SIFSIX-Cu-TPA (1.7),49 and ATU-Cu (1.2).33The high C2H2 adsorption capacity and moderate selectivity of JXNU-11(Fe2M) at ambient temperature reveal the greatly promising potential for C2H2/CO2 separation. To further investigate the C2H2/CO2 separation performance of JXNU-11(Fe2M), the breakthrough experiments were performed in practical separation processes for a C2H2/CO2 mixture (v∶v = 50∶50) at 298 K and 1 atm. The breakthrough experimental results show that CO2 was eluted first from the packed column, whereas C2H2 was retained in the bed for more than 120 and 108 min g−1 for JXNU-11(Fe2Ni) and JXNU-11(Fe2Co) (Figure 4a), respectively. The C2H2 breakthrough times for JXNU-11(Fe2M) rank among the leading MOFs ( Supporting Information Table S4). Moreover, the significant roll-up phenomenon of CO2 was observed in the breakthrough experiments, indicating a large proportion of previously adsorbed CO2 molecules can be replaced by the later fed C2H2 molecules. Such a phenomenon means that the C2H2 molecule competes better than CO2 for the binding sites of JXNU-11(Fe2M), highlighting the excellent separation efficiency of JXNU-11(Fe2M) for a C2H2/CO2 mixture. The breakthrough experiments were terminated when the concentrations of effluent gases stabilized. Figure 4 | C2H2 and CO2 separation performances. (a) Breakthrough curves of JXNU-11(Fe2M) at the flow of 2 mL min−1, 298 K, and 1 atm. Breakthrough curves of JXNU-11(Fe2Ni) at different temperatures (b) and different total flow rates at 298 K (c). Download figure Download PowerPoint The captured amounts for C2H2 during the 0∼tbreak time under the dynamic conditions are 4.8 and 4.3 mmol g−1 for JXNU-11(Fe2Ni) and JXNU-11(Fe2Co), respectively. The C2H2 captured amounts are remarkable and outperform all other MOFs, including the top-performing ZJU-74a (3.64 mmol g−1),42 SNNU-45 (3.5 mmol g−1),41 BSF-3 (2.9 mmol g−1),38 JCM-1 (2.2 mmol g−1),61 and FJU-90a (1.87 mmol g−1)36 (Figure 5 and Supporting Information Table S4). In addition, a long interval time of 55 min g−1 between C2H2 and CO2 breakthrough for JXNU-10(Fe2Ni) was obtained. Such an interval time stands out amongst MOFs and is only shorter than those of SNNU-45 (79 min g−1)41 and SIFSIX-Cu-TPA (69 min g−1).49 Furthermore, their practical separation potentials under different temperatures were evaluated. The C2H2 breakthrough times increased significantly with a decrease of temperature (Figure 4b and Supporting information Figure S9). The breakthrough times of C2H2 reached 146 (283 K) and 165 min g−1 (273 K) with a gas flow of 2 mL min−1. Figure 5 | Experimental breakthrough performance. Comparison of MOFs with top-high C2H2/CO2 breakthrough performance at 298 K and 1 atm. Download figure Download PowerPoint The separation performance of JXNU-11(Fe2M) with different gas flow rates at 298 K were also studied. With the gas flow of 2 mL min−1, the longest C2H2 breakthrough time and the largest amount of the adsorbed C2H2 were achieved for JXNU-11(Fe2M) (Figure 4c and Supporting Information Figure S10). JXNU-11(Fe2M) exhibited no appreciable changes in breakthrough times after three cycles of dynamic breakthrough experiments ( Supporting Information Figure S11), indicative of an excellent recycling separation capability. With the advantage of the modest adsorption heat of C2H2, JXNU-11(Fe2M) materials can be easily regenerated through purging the column with He gas at ambient temperature, as evidenced by the overlapped breakthrough curves ( Supporting Information Figure S11). Such results further indicate JXNU-11(Fe2M) are highly desirable microporous materials for C2H2/CO2 separation. Finally, the desorption experiments were carried out on JXNU-11(Fe2Ni). After reaching the breakthrough equilibrium, the adsorption column was purged with He gas (flow rate: 4 mL min−1) under ambient pressure and 303 K. As depicted in Figure 6a, the adsorbed CO2 molecules were released from the adsorption bed quickly. In contrast, the desorption of C2H2 gas was much slower. The productivity of C2H2 with over 95% purity was estimated from the desorption curve to be 1.98 mmol for 1 g of JXNU-11(Fe2Ni) (Figure 6b). Figure 6 | Breakthrough experiments. (a) and (b) Breakthrough curves and desorption curves of JXNU-11(Fe2Ni) based on the equimolar C2H2/CO2 mixture. Download figure Download PowerPoint To fully understand the underlying mechanism of the preferential adsorption of C2H2 over CO2 in JXNU-11(Fe2M), we performed comprehensive GCMC simulations to investigate the gas adsorption sites for JXNU-11(Fe2M). The calculated preferential binding sites of C2H2 are shown in Figures 7a and 7b. Four primary binding sites (sites I–IV) for C2H2 were found. As expected, the primary binding sites for C2H2 molecules are F and carboxylate O sites. For site I, site II, and site III, strong C−H···F hydrogen bonding notably occur between C2H2 and F atoms (H···F = 2.49–3.01 Å), which confirms the strong binding affinity of JXNU-11(Fe2M) toward C2H2. Compared with these sites, site IV exhibits strong C−H⋯O hydrogen bonds with a pair of carboxylate O atoms with the distances of 2.61 and 2.60 Å. These H⋯F and H⋯O distances are shorter than the sums of the corresponding van der Waals radii of hydrogen and fluorine (2.67 Å) or hydrogen and oxygen (2.72 Å) atoms, suggesting the substantial interactions. Moreover, the C≡C group of C2H2 interacts with the adjacent open metal sites through π⋯M interactions, and weakly interacts with the neighboring aromatic ring units of the framework through π⋯π interactions. Thus these multiple interactions between C2H2 molecules and the framework synergistically result in the remarkable affinity for C2H2. Therefore, the nanosized double-shell nests with many highly electronegative F and O atoms provide the polarized and fluorophilic pore spaces to accommodate C2H2. In contrast, the negatively charged F and O sites are not desirable sites for CO2. The presence of the repulsive interactions between the F/O atoms and the O atoms of CO2 leads to a weaker interaction between CO2 and the framework, which is consistent with the experimental results. The computational results gave an average binding energy of 31.8 kJ mol−1 for C2H2, which is much higher than that of 20.9 kJ mol−1 for CO2, further corroborating the stronger affinity toward C2H2 in the present framework. These calculated results clearly reveal that the rich F and O atoms as well as the open metal sites in the cage-in-cage structures are synergistically responsible for the preferential adsorption of C2H2 over CO2, thus affording the top-level C2H2/CO2 separation performance of JXNU-11(Fe2M). Figure 7 | Mechanism study. Calculated adsorption sites of C2H2 in JXNU-11(Fe2M). (a) Sites I and II; (b) Sites III and IV. Element key: Fe (dark yellow), Ni (teal), C (gray), F (bright green), and O (red) in JXNU-11(Fe2M); C (gold) and H (blue) in C2H2. The labeled distance is measured in Å. Download figure Download PowerPoint Conclusion We developed two robust trinuclear cluster-based MOFs (JXNU-11(Fe2M), M = Ni and Co) featuring distinctive nanosized cage-within-cage structures and highly polarized and fluorophilic pore environments. Such a distinctive cage-in-cage structure formed via interpenetration is composed of an inner octahedron and an outer large cuboctahedron. The double-shell nested cages in the MOFs provide the fascinating aesthetic features and the optimized pore spaces for trapping C2H2. The abundant F and O atoms of the organic ligands in JXNU-11(Fe2M) endow JXNU-11(Fe2M) with multiple binding sites for C2H2. The F and O atoms possessing high electronegativity in JXNU-11(Fe2M) pointing into the pore surfaces of the cages result in the highly dense electric charge density on the pore surfaces, which are the desirable sites for trapping C2H2 with positively charged H atoms. As a result, JXNU-11(Fe2M) shows a strong binding affinity to C2H2 over CO2. The nanosized double-shell cages in JXNU-11(Fe2M) afford the optimized pore spaces for trapping C2H2, leading to an efficient C2H2/CO2 separation. The practical breakthrough performance of JXNU-11(Fe2Ni) displayed the extraordinary breakthrough time for C2H2 gas and the benchmark C2H2-capture amount, the highest yet observed at ambient condition, confirming the best C2H2/CO2 separation performance of JXNU-11(Fe2M) materials. Thus we illustrate a useful strategy for the design of cage-in-cage structures in MOFs and develop an efficient approach for the construction of the highly polarized and fluorophilic pore cages in the uncommon fluorous MOFs. Supporting Information Supporting Information is available and includes detailed experimental methods, crystal structures, crystallographic data for JXNU-11(Fe2M), adsorption isotherms, column breakthrough experiments, and GCMC simulation. Conflict of Interest There is no conflict of interest to report. Funding Information This work is financially supported by the National Natural Science Foundation of China (nos. 22061022 and 21861020) and the Foundation for Academic and Technical Leaders of Major Disciplines of Jiangxi Province (no. 20204BCJL22043). References 1. Pässler P.; Hefner W.; Buckl K.; Meinass H.; Wernicke H. J.; Ebersberg G.; Müller R.; Bässler J.; Behringer H.; Mayer D.Ullmann’s Encyclopedia of Industrial Chemistry; Wiley-VCH: Weinheim, 2000. Google Scholar 2. Chien J. C. W.Polyacetylene: Chemistry, Physics, and Material Science. Academic: New York, 1984. Google Scholar 3. Granada A.; Karra S. B.; Senkan S. M.Conversion of Methane into Acetylene and Ethylene by the Chlorine-Catalyzed Oxidative-Pyrolysis (CCOP) Process. 1. Oxidative Pyrolysis of Chloromethane.Ind. Eng. Chem. Res.1987, 26, 1901–1905. Google Scholar 4. Kitagawa S.; Kitaura R.; Noro S. I.Functional Porous Coordination Polymers.Angew. Chem. Int. Ed.2004, 43, 2334–2375. Google Scholar 5. Furukawa H.; Cordova K. E.; Yaghi O. M.The Chemistry and Applications of Metal-Organic Frameworks.Science2013, 341, 1230444. Google Scholar 6. Bai Y.; Dou Y.; Xie L. H.; Rutledge W.; Li J. R.; Zhou H. C.Zr-Based Metal–Organic Frameworks: Design, Synthesis, Structure, and Applications.Chem. Soc. Rev.2016, 45, 2327–2367. Google Scholar 7. Stock N.; Biswas S.Synthesis of Metal-Organic Frameworks (MOFs): Routes to Various MOF Topologies, Morphologies, and Composites.Chem. Rev.2012, 112, 933–969. Google Scholar 8. Matsuda R.; Tsujino T.; Sato H.; Kubota Y.; Morishige K.; Takata M.; Kitagawa S.Temperature Responsive Channel Uniformity Impacts on Highly Guest-Selective Adsorption in a Porous Coordination Polymer.Chem. Sci.2010, 1, 315–321. Google Scholar 9. Kalmutzki M. J.; Hanikel N.; Yaghi O. M.Secondary Building Units as the Turning Point in the Development of the Reticular Chemistry of MOFs.Sci. Adv.2018, 4, eaat9180. Google Scholar 10. Ding M.; Flaig R. W.; Jiang H. L.; Yaghi O. M.Carbon Capture and Conversion Using Metal–Organic Frameworks and MOF-Based Materials.Chem. Soc. Rev, 2019, 48, 2783–2828. Google Scholar 11. Li B.; Chrzanowski M.; Zhang Y.; Ma S.Applications of Metal-Organic Frameworks Featuring Multi-Functional Sites.Coord. Chem. Rev.2016, 307, 106–129. Google Scholar 12. Yang L. F.; Qian S.; Wang X. B.; Cui X. L.; Chen B. L.; Xing H. B.Energy-Efficient Separation Alternatives: Metal–Organic Frameworks and Membranes for Hydrocarbon Separation.Chem. Soc. Rev
更多查看译文
关键词
fluorophilic pore surfaces,metal–organic frameworks,cage-in-cage
AI 理解论文
溯源树
样例
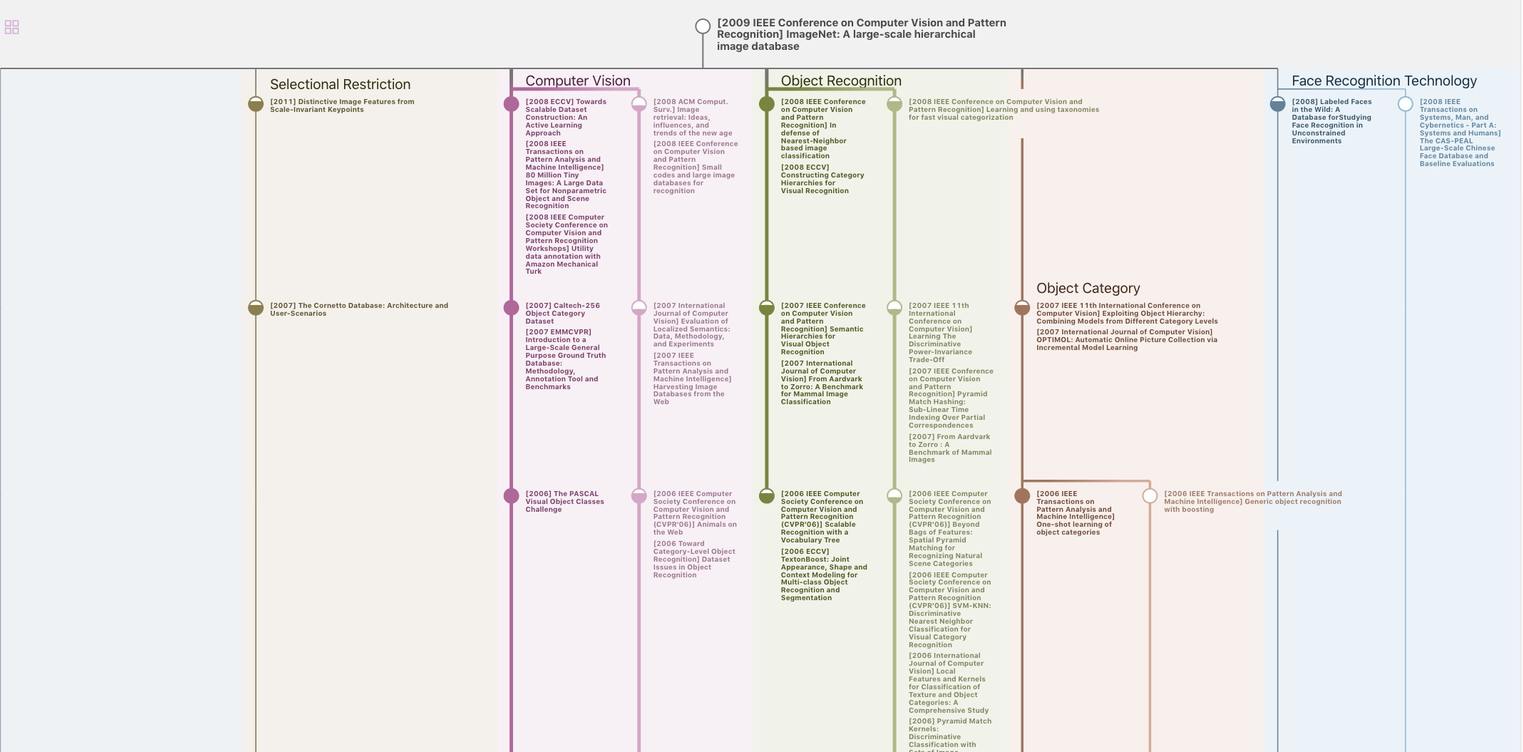
生成溯源树,研究论文发展脉络
Chat Paper
正在生成论文摘要