Nitrogen Reduction to Ammonia by a Phosphorus-Nitrogen PN 3 P-Mo(V) Nitride Complex: Significant Enhancement via Ligand Postmodification
CCS Chemistry(2023)
摘要
Open AccessCCS ChemistryCOMMUNICATIONS6 Dec 2022Nitrogen Reduction to Ammonia by a Phosphorus-Nitrogen PN3P-Mo(V) Nitride Complex: Significant Enhancement via Ligand Postmodification Delong Han, Priyanka Chakraborty, Mei-Hui Huang, Li Yang, Hao Huang, Théo P. Gonçalves, Abdul Hamid Emwas, Zhiping Lai, Jr-Hau He, Aleksander Shkurenko, Mohamed Eddaoudi and Kuo-Wei Huang Delong Han Division of Physical Science and Engineering, King Abdullah University of Science and Technology, Thuwal 23955 KAUST Catalysis Center, King Abdullah University of Science and Technology, Thuwal 23955 Google Scholar More articles by this author , Priyanka Chakraborty Division of Physical Science and Engineering, King Abdullah University of Science and Technology, Thuwal 23955 KAUST Catalysis Center, King Abdullah University of Science and Technology, Thuwal 23955 Google Scholar More articles by this author , Mei-Hui Huang Division of Physical Science and Engineering, King Abdullah University of Science and Technology, Thuwal 23955 KAUST Catalysis Center, King Abdullah University of Science and Technology, Thuwal 23955 Google Scholar More articles by this author , Li Yang Division of Physical Science and Engineering, King Abdullah University of Science and Technology, Thuwal 23955 KAUST Catalysis Center, King Abdullah University of Science and Technology, Thuwal 23955 Google Scholar More articles by this author , Hao Huang Division of Physical Science and Engineering, King Abdullah University of Science and Technology, Thuwal 23955 KAUST Catalysis Center, King Abdullah University of Science and Technology, Thuwal 23955 Google Scholar More articles by this author , Théo P. Gonçalves Division of Physical Science and Engineering, King Abdullah University of Science and Technology, Thuwal 23955 KAUST Catalysis Center, King Abdullah University of Science and Technology, Thuwal 23955 Google Scholar More articles by this author , Abdul Hamid Emwas Core Lab, King Abdullah University of Science and Technology, Thuwal 23955 Google Scholar More articles by this author , Zhiping Lai Division of Physical Science and Engineering, King Abdullah University of Science and Technology, Thuwal 23955 Advanced Membranes and Porous Materials Research Center, King Abdullah University of Science and Technology, Thuwal 23955 Google Scholar More articles by this author , Jr-Hau He Department of Materials Science and Engineering, City University of Hong Kong, Kowloon 999077 Google Scholar More articles by this author , Aleksander Shkurenko Division of Physical Science and Engineering, King Abdullah University of Science and Technology, Thuwal 23955 Advanced Membranes and Porous Materials Research Center, King Abdullah University of Science and Technology, Thuwal 23955 Google Scholar More articles by this author , Mohamed Eddaoudi Division of Physical Science and Engineering, King Abdullah University of Science and Technology, Thuwal 23955 Advanced Membranes and Porous Materials Research Center, King Abdullah University of Science and Technology, Thuwal 23955 Google Scholar More articles by this author and Kuo-Wei Huang *Corresponding author: E-mail Address: [email protected] Division of Physical Science and Engineering, King Abdullah University of Science and Technology, Thuwal 23955 KAUST Catalysis Center, King Abdullah University of Science and Technology, Thuwal 23955 Agency for Science, Technology, and Research, Institute of Materials Research and Engineeringand Institute of Sustainability for Chemicals, Energy and Environment, Singapore 138634 Google Scholar More articles by this author https://doi.org/10.31635/ccschem.022.202202385 SectionsSupplemental MaterialAboutAbstractPDF ToolsAdd to favoritesDownload CitationsTrack Citations ShareFacebookTwitterLinked InEmail Efforts to develop organometallic complexes for catalytic nitrogen reduction have seen significant progress in recent years. However, the strategies for improving the activity of the homogenous catalysts have mainly focused on alternating ligands and metals. Herein, we report that the activity and stability of a PN3P-Mo pincer complex ( 2) toward dinitrogen (N2) reduction were greatly enhanced through postmodification of the PN3P pincer framework of its parent complex ( 1). A high ratio of NH3/Mo (3525) was achieved in the presence of SmI2 as a reductant. In sharp contrast, 1 only afforded an NH3/Mo ratio of 21. Moreover, when supported by an anionic pincer ligand, 2 furnished a high oxidation state Mo(V)-nitride complex via N2 cleavage as a plausible key intermediate in the catalytic process, suggesting a catalytic cycle that may involve different oxidation states (II/V) from those with 10-π electron configuration in the literature. Download figure Download PowerPoint Introduction The annual production of approximately 180 million tons of NH3 consumes roughly 1–2% of the global energy supply.1,2 It is thus desirable to develop alternative routes to produce NH3 under milder conditions in an efficient and environmentally friendly manner. Organometallic complexes have been investigated for decades in search of potent catalysts for nitrogen fixation.3–10 After the breakthrough by Yandulov and Schrock in 2003 to successfully transform N2 into NH3 with cobaltocene as the reducing agent and a Mo complex as a catalyst under ambient conditions (Figure 1a),11 various organometallic complexes, such as Fe,12–16 Mo,17–19 Co,20 V,21 and Re,22–24 were employed for the investigation of N2 reduction reactions (NRRs). Among these different combinations of metal-ligand platforms, Nishibayashi and co-workers first demonstrated that pincer-type Mo complexes could serve as catalysts under highly reductive conditions in the presence of proper proton sources (Figure 1b),25 thereby stimulating more research on pincer complexes for such studies (Figure 1).20,26–31 However, despite significant contributions by the scientific community, exploring potential catalysts has been restricted by conventional synthetic methods. Figure 1 | Selected Mo complexes employed in the NRR. Download figure Download PowerPoint We have developed a series of PN3(P) pincer complexes where a more acidic N–H spacer, compared with the C–H counterparts,32–34 makes the transformation between dearomatized and aromatized forms readily accessible for hydrogenative and dehydrogenative reactions.35,36 Very interestingly, these complexes allow the postmodification of the ligand to afford a new class of second-generation PN3P pincer complexes that are difficult to synthesize via conventional synthetic methods (Scheme 1). This new class of PN3P complexes completely lose the properties of reversible dearomatization and rearomatization, but the ligands appear to be more electron-donating toward the metal center and may stabilize metal centers with higher oxidation states.37,38 The feature of the ligand modification may provide an opportunity to explore potential catalysts with high oxidation states.39,40 In the case of Mo catalysts for NRR, previous studies suggest two pathways involving Mo complexes containing terminal or bridging N2 ligand(s).41–44 Higher catalytic activities were observed when a bridging N2 was cleaved into nitride with a Mo center possessing a positive charge.17,45 Therefore, the anionic second-generation PN3P ligand may offer some advantage over the parent neutral ligand. Scheme 1 | Synthesis of Mo pincer complexes. Download figure Download PowerPoint During our study on the influences and potential merits of postmodification on NRR by comparing Mo complex 2 with its parent complex 1, the Nishibayashi group reported a new nitrogen reduction combination of SmI2 as reductant and ethylene glycol as a proton source. An N-heterocyclic carbene-based Mo pincer complex catalyzed the transformation of N2 into NH3 with high activity (4350 NH3/equiv, Figure 1e) under the new condition via a proton-coupled electron-transfer (PCET) process.45 The new N2 reduction condition offers us an appropriate platform to examine the influences of postmodification on the catalytic activity under mild conditions. Herein, we report a second-generation PN3P-Mo pincer complex ( 2) prepared via postmodification of the PN3P-Mo precursor ( 1)17 as a potent precatalyst for N2 reduction to NH3. Meanwhile, in contrast to the neutral pincer ligands, such as PNP and PPP, where only the formation of pincer Mo(IV) nitride complexes was observed (Figure 2a),17,26 a higher oxidation Mo(V) nitride complex ( 3), derived from 2, is the catalytically active species (Figure 2b). Figure 2 | Pincer Mo-nitride catalysts formed during N2 reduction. Download figure Download PowerPoint Results and Discussion Complex 1 was synthesized according to the literature procedure.46 As expected, the PN3P ligand adopts a meridional coordination mode with three chloride ions connected to the Mo center on one plane vertical to that of the pincer ligand (Figure 3, left). Complex 2 was prepared by treating 1 with excess amounts of t-BuOK and ethyl iodide (Scheme 1),39 and its structure was confirmed by the X-ray crystallographic analysis (Figure 3, right).a In the molecular structure of 2, besides the tridentate ligand (one amide N and two phosphine P atoms), the Mo center is also coordinated by one iodide and one oxo ligand. The pincer ligand was modified by the addition of three ethyl groups to the pyridine backbone. The (t-Bu2P)2Mo(O)I moiety was disordered over two positions (see Supporting Information for details). Complex 2 could also be generated by replacing t-BuOK with KH as the base under otherwise similar conditions, but no product was found when toluene was used as the solvent, suggesting that the Mo metal center may abstract oxygen from tetrahydrofuran (THF) to form the Mo=O functionality with the formation of 1-butene ( Supporting Information Figure S3). Figure 3 | Molecular structure of 1 (left) and 2 (right). Thermal ellipsoids are shown at the 30% probability level. Only disordered atoms of part A are shown. For clarity, only selected atoms are labeled and all the solvent molecules and hydrogen atoms (except that of NH) were omitted. Selected bonds (Å): Mo1–N1 2.226(2), Mo1–P1 2.606(1), Mo1–P2 2.583(1), Mo1–Cl1 2.417(1), Mo1–Cl2 2.450(1), Mo1–Cl3 2.391(1), N2–C1 1.377(3), N3–C5 1.378(3). Selected angles (°): P1–Mo1–P2 152.13(2), N1–Mo1–Cl2 166.75(6), Cl1–Mo1–Cl3 168.78(3). For 2, Selected bonds (Å): Mo1A–N1 2.139(2), Mo1A–P1A 2.469(1), Mo1A–P2A 2.467(1), Mo1A–O1A 1.658(2), Mo1A–I1A 2.770(1), N2−C1 1.291(4), N3–C5 1.286(4). Selected angles (°): P1A–Mo1A–P2A 146.39(4), N1–Mo1A–I1A 146.21(6). Download figure Download PowerPoint Interestingly, the N1–Mo1 bond distance in 1 (2.226(2) Å) is longer than that in 2 (2.139(2) Å), suggesting that the amide ligand may have a stronger electron-donating ability toward the Mo center. Moreover, the N2–C1 and N3–C5 bond lengths in 1 (1.377(3) and 1.378(3) Å, respectively) are also longer than those in 2 (1.291(4) and 1.286(4) Å, respectively), which agrees well with the double bond characteristics of 2. Furthermore, the aromaticity of the six-numbered ring was completely lost with only one double bond remaining (C3–C4 1.329(4) Å), and the bond distance of Mo1A–O1A in 2 displayed a typical double bond character (1.658(2) Å).47 Moreover, the angle P1–Mo1–P2 became smaller: from 152.13(2) in 1 to 146.39(4) in 2. At the beginning of the NRR study, the bases and temperatures were optimized by employing 2 as the catalyst with ethylene glycol as a proton source and SmI2 as a reductant (Table 1).48 We found that the reaction at 50 °C employing t-BuOK rather than KOH after reaction under our condition afforded NH3 in the best yield of 94% (Table 1, entry 4).b In contrast, when 1 was applied as the catalyst under the same condition, only NH3 in 33% yield was achieved (Table 2, entry 1), significantly lower than that of 2. Although 2 is derived from 1, its structure and activity are significantly changed. Next, nitrogen reduction was investigated with SmI2, 2, and various proton sources. H2O as a proton source furnished NH3 in 79% yield (Table 2, entry 2). Table 1 | Optimization of the Reaction Conditions for NRR.a Entry Base Temperature NH3 Yield (%)c NH3/equivd H2 Yield (%)c 1 No r.t. 0 0 0 2 No 50 °C 7 4 4 3 KOHb 50 °C 71 42 4 4 tBuOK 50 °C 94 56 4 5 tBuOK 40 °C 86 52 0 6 tBuOK r.t. 73 44 0 7 tBuOKe 50 °C 71 42 0 8 tBuOKf 50 °C 91 55 0 aReaction condition: 2 (2 mg, 2.6 μmol), SmI2 (470 μmol, 180 equiv based on 2), ethylene glycol (470 μmol, 180 equiv based on the catalyst), N2 atmosphere, 3 h. t-BuOK (8.9 mmol) was added after the reaction. H2 was detected by an online gas chromatography (GC) equipped with a thermal conductivity detector (TCD), and a flame ionization detector (FID) with a mechanizer was used for H2 quantification. bKOH 30 wt %, 10 mL. cThe yield was calculated based on the reductant. dNH3/equiv was calculated based on the Mo complex. Each reaction was repeated at least twice. e40 min. f1 h. Table 2 | Catalytic N2 Reduction to Ammonia with Different Proton Sources and Reductants.a Entry Catalyst Proton Source NH3 Yield (%)b NH3/equivc H2 Yield (%)b 1 1 HO(CH2)2OH 33 21 20 2 2 H2O 79 47 4 3 2 CH3OH 93 56 1 4 2 CH3CH2OH 48 30 4 5 2 CF3CH2OH 5 3 22 6 2 (CH3)2CHOH 6 4 48 7 2 2,3-Butanediol 12 7 47 8 2 1,2-Cyclohexanediol 2 1 11 9d 2 HO(CH2)2OH 83 ± 3 540 ± 18 10 ± 3 10d 2 CH3OH 39 228 2 11e 2 HO(CH2)2OH 59 ± 1 3525 ± 61 13 ± 2 12f 1 [Lut-H][OTf] 2 1 0 13f 2 [Lut-H][OTf] 11 4 0 14g 2 HO(CH2)2OH 3 2 0 15h 2 HO(CH2)2OH 3 2 0 aReaction condition: Catalyst (2.6 μmol), SmI2 (470 μmol, 180 equiv based on the catalyst) as the reductant, proton source (470 μmol in dihydric alcohol, 940 μmol in monohydric alcohol, 180 and 360 equiv, respectively, based on the catalyst) in 5 mL THF was heated at 50 °C for 3 h. t-BuOK (8.9 mmol) was added after reaction. Each reaction condition was repeated at least twice. H2 was detected by online GC equipped with a TCD, and FID with a mechanizer was used for H2 quantification. bThe yield was calculated based on the reductant. cNH3/equiv was calculated based on the Mo complex. d0.26 μmol of catalyst 2 was used. e0.026 μmol of catalyst 2 was used, overnight. Repeated four times. fCatalyst (4.6 μmol), KC8 as reductant (100 equiv based on the catalyst), [Lut-H][OTf] as proton source (120 equiv based on the catalyst), room temperature. gKC8 was used as reductant (470 μmol), room temperature. hCp2Co (Cp, cyclopentadienyl) was used as reductant (470 μmol). An NH3 yield of up to 93% was obtained when methanol (MeOH) was employed as a proton source (Table 2, entry 3). Intriguingly, the yields decreased to 48% and 6% when ethanol and 2-propanol were used, respectively (Table 2, entries 4 and 6). Moreover, with a strong electron-withdrawing group in the alcohol, the yield became even lower (5%, Table 2, entry 5). A similar trend was observed when 2,3-butanediol and 1,2-cyclohexanediol were utilized; only 12% and 2% NH3 were produced, respectively (Table 2, entries 7 and 8). It is noteworthy that decreasing the catalyst loading of 2 from 2.6 to 0.26 μmol still gave an excellent yield of 83% for the NH3 production (Table 2, entry 9). However, when MeOH was employed, a lower yield of 39% was observed. Further reducing the catalyst loading to 0.026 μmol resulted in NH3 formation in 59% yield with 3525 NH3/equiv (Table 2, entry 11). Furthermore, 2 was also tested using [Lut-H][OTf] as a proton source with a more potent reductant, KC8, producing NH3 in 11% yield (Table 2, entry 13). When employing 1 as the catalyst under otherwise identical conditions, NH3 was observed in only 2% yield (Table 2, entry 12). Notably, in all cases, the yields of NH3 by 2 were consistently higher than those by 1. Employing KC8 or Cp2Co as the reductant and ethylene glycol as the proton source resulted in only 3% yield of NH3 (Table 2, entries 14 and 15), implying that the combination of other stronger reductants or a reductant with similar reducing ability and alcohol is not suitable for the N2 reduction compared to the optimized conditions using SmI2 and ethylene glycol or MeOH. 15N2 reduction using 2 as the catalyst and the same procedure gave an isotopically labeled 15NH3 as identified by the 1H NMR spectrum ( Supporting Information Figure S1). The result confirmed that N2 gas is the source of NH3 under our reaction conditions. A larger-scale experiment was carried out, and approximately 383 mg of NH4Cl was isolated in 81% yield (see Supporting Information Section 1.2). As the formation of a Mo-nitride complex may occur by the cleavage of an N2-bridged intermediate,41,49 the fate of complex 2 under the reduction condition was investigated. When 2 was treated with a stoichiometric amount of SmI2 and MeOH under N2 at 50 °C, conversion was observed with the formation of a new complex as an inseparable mixture ( Supporting Information Scheme S6), and no reaction was observed without a proton source. To identify whether the new complex was a Mo-nitride complex, complex 3 was synthesized by mixing NaI or SmI2 with 3-Cl (Figure 5) prepared from the PN3P ligand, Mo salt, and Me3SiN3 (Scheme 2).50 The infrared spectra of complex 3 and the reaction mixture showed similar characteristic peaks of Mo≡N ( Supporting Information Figure S4). Thus, the formation of 3 from the coordinately unsaturated Mo complex 2 under the catalytic conditions as the active catalytic species is strongly supported. Additionally, crystals of both 3-Cl and 3 were obtained for single-crystal X-ray analysis. Interestingly, the cell parameters of complex 3 are similar to those of 2, but both 3-Cl and 3 displayed distinct properties from those of 2 in terms of magnetism, NMR, mass, elemental analysis, and color ( Supporting Information Table S4). The structure of 3 exhibits a distorted square pyramidal structure (Figure 4); 3 completely loses the aromaticity, and the N2–C1 and N3–C5 bond lengths of the two arms become shorter from 1.370(5) Å and 1.375(5) Å in 4-I to 1.304(7) Å and 1.286(7) Å in 3, respectively, which fall into the range of a C=N double bond. Additionally, the angle of P1–Mo1–P2 becomes smaller from 151.06(3) to 148.63(6)°. The bond distance of N1–Mo1 declines from 2.199(3) Å to 2.190(4) Å, and that of Mo1–N4 decreases from 1.637(4) Å to 1.629(5) Å. It is also important to note that the bond distance of the Mo-nitride bond in 3-Cl is 1.644 Å, slightly longer than 1.635 Å in 4, indicating that the postmodified ligand system may have a better electron-donating ability ( Supporting Information Table S4). When we subjected 2 to the best reaction condition, the unmodified Mo nitride complex 4 only afforded NH3 in 13% yield, even though 4 shares the same Mo≡N core as 3-Cl and 3. In contrast, when 3 was employed as a catalyst for NRR under the optimized condition as 2, NH3 was formed in 90% yields ( Supporting Information Table S1). Figure 4 | Molecular structure of 3. Thermal ellipsoids are shown at the 30% probability level. Only disordered atoms of part A are shown. For clarity, only selected atoms are labeled and all hydrogen atoms were omitted. Selected bonds (Å): N2–C1 1.304(7), N3–C5 1.286(7), Mo1A–N1 2.190(4), Mo1A–N1A 1.629(5), Mo1A–P1A 2.513(2), Mo1A–P2A 2.514(2), Mo1A–I1A 2.720(1). Selected angles (°): P1A–Mo1A–P2A 148.63(6), N1–Mo1A–I1A 152.2(1). Download figure Download PowerPoint Figure 5 | Molecular structure of 3-Cl (right) and 4-I (left). Thermal ellipsoids are shown at the 30% probability level. Only disordered atoms of part A are shown. For clarity, only selected atoms are labeled and hydrogen atoms (except that of NH) were omitted. For 3-Cl, selected bonds (Å): N2–C1 1.289(9), N3–C5 1.297(8), Mo1A–N4A 1.644(7), Mo1A–N1 2.196(5), Mo1A–Cl1A 2.368(2), Mo1A–P2A 2.524(3), Mo1A–P1A 2.504(3). Selected angles (°): N1–Mo1A–Cl1A 146.9(2), P1A–Mo1A–P2A 148.42(9). For 4-I, except H2 and H3, hydrogen atoms and non-coordinated iodide anion are omitted for clarity. Selected bonds (Å): N2–C1 1.370(5), N3–C5 1.375(5), Mo1–N1 2.199(3), Mo1–P1 2.519(1), Mo1–P2 2.517(1), Mo1–I1 2.702(4), Mo1–N4 1.637(4). Selected angles (°): P1–Mo1–P2 151.06(3), N1–Mo1–I1 155.66(8). Download figure Download PowerPoint To gain further insights into the influence of the ligand postmodification, stoichiometric reactions of 1 with SmI2 were carried out. Interestingly, when supported by a PN3P ligand, 1 was transformed into a PN3P-Mo nitride complex ( 4-I) with SmI2 under N2 (Scheme 3), in sharp contrast to the formation of a PNP-Mo(IV) in an analogous reaction,45 which was also distinct from the reduction with KC8 and [Lut-H][OTf] where the Mo center was reduced to 0 with terminal N2 ligands.46 4-I possesses a Mo(V) center, structurally similar to complex 4 (Figure 5). However, 4-I was only obtained in 20% yield in the stoichiometric reaction with insoluble precipitates. The fact that there were no more other Mo pincer complexes identified from the reaction mixture suggests the poor stability of 1 under the NRR condition. These observations indicate that the second-generation PN3P ligand affords a significantly improved stability of the corresponding Mo pincer complexes, resulting in the excellent catalytic performance of 2 and 3 in the NRR. Scheme 2 | Syntheses of Mo pincer complexes 3 and 4. Download figure Download PowerPoint Scheme 3 | Stoichiometric reactions for complex 1. Download figure Download PowerPoint We propose a plausible catalytic cyclic according to these experimental observations and literature reports (Scheme 4 and Supporting Information Section 3).17,30,51 First, the oxo ligand dissociates from the reduced Mo center after 2 receives electrons and protons and forms reactive intermediate PN3P-MoII-I (MoI, Supporting Information Scheme S7). Two of MoI molecules are then connected by an N2 bridge to give a penta-coordinated bimetallic intermediate Int, followed by the cleavage of the N2 bridge into two complex 3.17,26,30,51–53 After that, the Mo center was reduced by SmI2 with the nitride ligand protonated to NH3 in a series of PCET reactions to regenerate Int in the presence of N2. The oxidation states of second-generation PN3P Mo complexes change between II and V in the catalytic process (between 3 and Int), in contrast to the I and IV cycle reported in the conventional Mo pincer counterparts.45 Of note is the Mo(V) complexes were reported before,43,50,51,54 and the N2 cleavage by Mo(II) to the corresponding Mo(V)≡N complex was also recently demonstrated.25,28 The experimental results and calculations indicate that the reaction may proceed via a II/V cycle, but we cannot completely rule out the involvement of other oxidation states that were not observed experimentally.3 Scheme 4 | A plausible mechanism for N2 reduction by the PN3P-Mo complex. Download figure Download PowerPoint Conclusion We have prepared a second-generation PN3P-Mo pincer complex 2 via the postmodification from complex 1 for the N2 reduction under various conditions. The catalytic activity and stability of the resulting pincer Mo complexes ( 2 and 3) are significantly influenced by the ligand structures, especially when compared with those “unmodified” counterparts ( 1 and 4) using SmI2 as the reductant: 2 and 3 with yields of >90%, but only 33% and 13% yields for 1 and 4, respectively; a high number of NH3/Mo of 3525 was achieved. Supported by the anionic modified ligand, the second-generation PN3P Mo complex 2 was converted to a Mo(V) nitride intermediate during the catalytic reaction by cleaving N2. Compared with much-less active Mo(V) nitride complexes formed from 1 under similar conditions, this postmodification resulted in high activity of the Mo center in the catalytic cycle. These observations not only indicate a broader working range of oxidation states of Mo for nitrogen reduction but also again demonstrate a profound effect of the ligand modification. The combination of SmI2 with ethylene glycol or MeOH affords excellent yields for the N2 reduction to NH3 in our system. The postmodification resulted in a significant enhancement of the catalytic activities of the PN3P-Mo complexes. Investigation on the synthetic applications for nitrogen fixation using complexes 2 and 3 is ongoing and will be reported in due course. Footnotes a According to our experience on the known post modified pincer complexes, a small amount of by product is also generated in which two ethyl groups are connected to the carbon next to C1. Highly pure 2 was obtained via recrystallization. b The literature shows that the resulting Sm complex gives a Sm alkoxide dimer after the reaction, suggesting that the use of alkoxide or hydroxide is needed to destroy the Sm-NH3 complexes to release NH3. See refs 45 and 48. Supporting Information Supporting Information is available and includes experimental section and supporting figures. Conflict of interest The authors declare no conflict of interest. Acknowledgments We are grateful for the service of NOOR 2, Shaheen 2 High-Performance Computing Facilities, and financial support from King Abdullah University of Science and Technology (KAUST). We acknowledge Dr. Xingzhu Chen and Amy Perez for the graphic design. References 1. Gruber N.; Galloway J. N.An Earth-System Perspective of the Global Nitrogen Cycle.Nature2008, 451, 293–296. Google Scholar 2. Giddey S.; Badwal S. P. S.; Munnings C.; Dolan M.Ammonia as a Renewable Energy Transportation Media.ACS Sustain. Chem. Eng.2017, 5, 10231–10239. Google Scholar 3. Creutz S. E.; Peters J. C.Catalytic Reduction of N2 to NH3 by an Fe–N2 Complex Featuring a C-Atom Anchor.J. Am. Chem. Soc.2014, 136, 1105–1115. Google Scholar 4. Hirotsu M.; Fontaine P. P.; Epshteyn A.; Sita L. R.Dinitrogen Activation at Ambient Temperatures: New Modes of H2 and PhSiH3 Additions for an “End-On-Bridged” [Ta(IV)]2(μ-η:η1-N2) Complex and for the Bis(μ-nitrido) [Ta(V)(μ-N)]2 Product Derived from Facile N N Bond Cleavage.J. Am. Chem. Soc.2007, 129, 9284–9285. Google Scholar 5. Guru M. M.; Shima T.; Hou Z.Conversion of Dinitrogen to Nitriles at a Multinuclear Titanium Framework.Angew. Chem. Int. Ed.2016, 55, 12316–12320. Google Scholar 6. Camp C.; Pécaut J.; Mazzanti M.Tuning Uranium–Nitrogen Multiple Bond Formation with Ancillary Siloxide Ligands.J. Am. Chem. Soc.2013, 135, 12101–12111. Google Scholar 7. Falcone M.; Barluzzi L.; Andrez J.; Fadaei Tirani F.; Zivkovic I.; Fabrizio A.; Corminboeuf C.; Severin K.; Mazzanti M.The Role of Bridging Ligands in Dinitrogen Reduction and Functionalization by Uranium Multimetallic Complexes.Nat. Chem.2019, 11, 154–160. Google Scholar 8. Wang K.; Deng Z.-H.; Xie S.-J.; Zhai D.-D.; Fang H.-Y.; Shi Z.-J.Synthesis of Arylamines and N-Heterocycles by Direct Catalytic Nitrogenation Using N2.Nat. Commun.2021, 12, 248–255. Google Scholar 9. Fajardo J.; Peters J. C.Catalytic Nitrogen-to-Ammonia Conversion by Osmium and Ruthenium Complexes.J. Am. Chem. Soc.2017, 139, 16105–16108. Google Scholar 10. Mo Z.; Shima T.; Hou Z.Synthesis and Diverse Transformations of a Dinitrogen Dititanium Hydride Complex Bearing Rigid Acridane-Based PNP-Pincer Ligands.Angew. Chem. Int. Ed.2020, 59, 8635–8644. Google Scholar 11. Yandulov D. V.; Schrock R. R.Catalytic Reduction of Dinitrogen to Ammonia at a Single Molybdenum Center.Science2003, 301, 76–78. Google Scholar 12. Schild D. J.; Peters J. C.Light Enhanced Fe-Mediated Nitrogen Fixation: Mechanistic Insights Regarding H2 Elimination, HER, and NH3 Generation.ACS Catal.2019, 9, 4286–4295. Google Scholar 13. Kuriyama S.; Arashiba K.; Nakajima K.; Matsuo Y.; Tanaka H.; Ishii K.; Yoshizawa K.; Nishibayashi Y.Catalytic Transformation of Dinitrogen into Ammonia and Hydrazine by Iron-Dinitrogen Complexes Bearing Pincer Ligand.Nat. Commun.2016, 7, 12181–12190. Google Scholar 14. Matson B. D.; Peters J. C.Fe-Mediated HER vs N2RR: Exploring Factors That Contribute to Selectivity in P3EFe(N2) (E = B, Si, C) Catalyst Model Systems.ACS Catal.2018, 8, 1448–1455. Google Scholar 15. Chalkley M. J.; Del Castillo T. J.; Matson B. D.; Roddy J. P.; Peters J. C.Catalytic N2-to-NH3 Conversion by Fe at Lower Driving Force: A Proposed Role for Metallocene-Mediated PCET.ACS Cent. Sci.2017, 3, 217–223. Google Scholar 16. Sekiguchi Y.; Kuriyama S.; Eizawa A.; Arashiba K.; Nakajima K.; Nishibayashi Y.Synthesis and Reactivity of Iron-Dinitrogen Complexes Bearing Anionic Methyl- and Phenyl-Substituted Pyrrole-Based PNP-Type Pincer Ligands Toward Catalytic Nitrogen Fixation.Chem. Commun.2017, 53, 12040–12043. Google Scholar 17. Arashiba K.; Eizawa A.; Tanaka H.; Nakajima K.; Yoshizawa K.; Nishibayashi Y.Catalytic Nitrogen Fixation via Direct Cleavage of Nitrogen–Nitrogen Triple Bond of Molecular Dinitrogen Under Ambient Reaction Conditions.Bull. Chem. Soc. Jpn.2017, 90, 1111–1118. Google Scholar 18. Kuriyama S.; Arashiba K.; Nakajima K.; Tanaka H.; Yoshizawa K.; Nishibayashi Y.Azaferrocene-Based PNP-Type Pincer Ligand: Synthesis of Molybdenum, Chromium, and Iron Complexes and Reactivity Toward Nitrogen Fixation.Eur. J. Inorg. Chem.2016, 4856–4861. Google Scholar 19. Hoffman B. M.; Lukoyanov D.; Yang Z.-Y.; Dean D. R.; Seefeldt L. C.Mechanism of Nitrogen Fixation by Nitrogenase: The Next Stage.Chem. Rev.2014, 114, 4041–4062. Google Scholar 20. Kuriyama S.; Arashiba K.; Tanaka H.; Matsuo Y.; Nakajima K.; Yoshizawa K.; Nishibayashi Y.Direct Transformation of Molecular Dinitrogen into Ammonia Catalyzed by Cobalt Dinitrogen Complexes Bearing Anionic PNP Pincer Ligands.Angew. Chem. Int. Ed.2016, 55, 14291–14295. Google Scholar 21. Imayoshi R.; Nakajima K.; Nishibayashi Y.Vanadium-Catalyzed Reduction of Molecular Dinitrogen into Silylamine Under Ambient Reaction Conditions.Chem. Lett.2017, 46, 466–468. Google Scholar 22. Lindley B. M.; van Alten R. S.; Finger M.; Schendzielorz F.; Würtele C.; Miller A. J. M.; Siewert I.; Schneider S.Mechanism of Chemical and Electrochemical N2 Splitting by a Rhenium Pincer Complex.J. Am. Chem. Soc.2018, 140, 7922–7935. Google Scholar 23. Klopsch I.; Finger M.; Würtele C.; Milde B.; Werz D. B.; Schneider S.Dinitrogen Splitting and Functionalization in the Coordination Sphere of Rhenium.J. Am. Chem. Soc.2014, 136, 6881–6883. Google Scholar 24. Klopsch I.; Kinauer M.; Finger M.; Würtele C.; Schneider S.Conversion of Dinitrogen into Acetonitrile Under Ambient Conditions.Angew. Chem. Int. Ed.2016, 55, 4786–4789. Google Scholar 25. Arashiba K.; Miyake Y.; Nishibayashi Y.A Molybdenum Complex Bearing PNP-Type Pincer Ligands Leads to the Catalytic Reduction of Dinitrogen into Ammonia.Nat. Chem.2011, 3, 120–125. Google Scholar 26. Liao Q.; Cavaillé A.; Saffon-Merceron N.; Mézailles N.Direct Synthesis of Silylamine from N2 and a Silane: Mediated by a Tridentate Phosphine Molybdenum Fragment.Angew. Chem.2016, 128, 11378–11382. Google Scholar 27. Keane A. J.; Farrell W. S.; Yonke B. L.; Zavalij P. Y.; Sita L. R.Metal-Mediated Production of Isocyanates, R3EN=C=O from Dinitrogen, Carbon Dioxide, and R3ECl.Angew. Chem. Int. Ed.2015, 54, 10220–10224. Google Scholar 28. Kendall A. J.; Johnson S. I.; Bullock R. M.; Mock M. T.Catalytic Silylation of N2 and Synthesis of NH3 and N2H4 by Net Hydrogen Atom Transfer Reactions Using a Chromium P4 Macrocycle.J. Am. Chem. Soc.2018, 140, 2528–2536. Google Scholar 29. Sekiguchi Y.; Arashiba K.; Tanaka H.; Eizawa A.; Nakajima K.; Yoshizawa K.; Nishibayashi Y.Catalytic Reduction of Molecular Dinitrogen to Ammonia and Hydrazine Using Vanadium Complexes.Angew. Chem. Int. Ed.2018, 57, 9064–9068. Google Scholar 30. Hebden T. J.; Schrock R. R.; Takase M. K.; Müller P.Cleavage of Dinitrogen to Yield a (t-BuPOCOP)molybdenum(IV) Nitride.Chem. Commun.2012, 48, 1851–1853. Google Scholar 31. Wickramasinghe L. A.; Ogawa T.; Schrock R. R.; Müller P.Reduction of Dinitrogen to Ammonia Catalyzed by Molybdenum Diamido Complexes.J. Am. Chem. Soc.2017, 139, 9132–9135. Google Scholar 32. Hu P.; Ben-David Y.; Milstein D.General Synthesis of Amino Acid Salts from Amino Alcohols and Basic Water Liberating H2.J. Am. Chem. Soc.2016, 138, 6143–6146. Google Scholar 33. Das U. K.; Kumar A.; Ben-David Y.; Iron M. A.; Milstein D.Manganese Catalyzed Hydrogenation of Carbamates and Urea Derivatives.J. Am. Chem. Soc.2019, 141, 12962–12966. Google Scholar 34. Gunanathan C.; Milstein D.Metal–Ligand Cooperation by Aromatization-Dearomatization: A New Paradigm in Bond Activation and “Green” Catalysis.Acc. Chem. Res.2011, 44, 588–602. Google Scholar 35. Li H.; Gonçalves T. P.; Lupp D.; Huang K.-W.PN3(P)-Pincer Complexes: Cooperative Catalysis and Beyond.ACS Catal.2019, 9, 1619–1629. Google Scholar 36. Li H.; Zheng B.; Huang K.-W.A New Class of PN3-Pincer Ligands for Metal-Ligand Cooperative Catalysis.Coord. Chem. Rev.2015, 293-294, 116–138. Google Scholar 37. Yao C.; Wang X.; Huang K.-W.Nitrogen Atom Transfer Mediated by a New PN3P-Pincer Nickel Core via a Putative Nitrido Nickel Intermediate.Chem. Commun.2018, 54, 3940–3943. Google Scholar 38. Yao C.; Zhang T.; Zhou C.; Huang K.-W.Interrogating the Steric Outcome During H2 Heterolysis: In-Plane Steric Effects in the Regioselective Protonation of the PN3P-Pincer Ligand.Dalton Trans.2019, 48, 12817–12821. Google Scholar 39. Wang X.; Yao L.; Pan Y.; Huang K.-W.Synthesis of Group 10 Metal Complexes with a New Unsymmetrical PN3P-Pincer Ligand Through Ligand Post-Modification: Structure and Reactivity.J. Organomet. Chem.2017, 845, 25–29. Google Scholar 40. Pan Y.; Guan C.; Li H.; Chakraborty P.; Zhou C.; Huang K.-W.CO2 Hydrogenation by Phosphorus-Nitrogen PN3P-Pincer Iridium Hydride Complexes: Elucidation of the Deactivation Pathway.Dalton Trans.2019, 48, 12812–12816. Google Scholar 41. Yamout L. S.; Ataya M.; Hasanayn F.; Holland P. L.; Miller A. J. M.; Goldman A. S.Understanding Terminal Versus Bridging End-on N2 Coordination in Transition Metal Complexes.J. Am. Chem. Soc.2021, 143, 9744–9757. Google Scholar 42. Chatt J.; Pearman A. J.; Richards R. L.The Reduction of Mono-Coordinated Molecular Nitrogen to Ammonia in a Protic Environment.Nature1975, 253, 39–40. Google Scholar 43. Tanaka H.; Arashiba K.; Kuriyama S.; Sasada A.; Nakajima K.; Yoshizawa K.; Nishibayashi Y.Unique Behaviour of Dinitrogen-Bridged Dimolybdenum Complexes Bearing Pincer Ligand Towards Catalytic Formation of Ammonia.Nat. Commun.2014, 5, 3737–3746. Google Scholar 44. Engesser T. A.; Kindjajev A.; Junge J.; Krahmer J.; Tuczek F.A Chatt-Type Catalyst with One Coordination Site for Dinitrogen Reduction to Ammonia.Chem.-Eur. J.2020, 26, 14807–14812. Google Scholar 45. Ashida Y.; Arashiba K.; Nakajima K.; Nishibayashi Y.Molybdenum-Catalysed Ammonia Production with Samarium Diiodide and Alcohols or Water.Nature2019, 568, 536–540. Google Scholar 46. Stucke N.; Krahmer J.; Näther C.; Tuczek F.Molybdenum Complexes Supported by PN3P Pincer Ligands: Synthesis, Characterization, and Application to Synthetic Nitrogen Fixation.Eur. J. Inorg. Chem.2018, 2018, 5108–5116. Google Scholar 47. Sun Z.; Zhao Y.; Prior T. J.; Elsegood M. R. J.; Wang K.; Xing T.; Redshaw C.Mono-Oxo Molybdenum(V) and Tungsten(VI) Complexes Bearing Chelating Aryloxides: Synthesis, Structure and Ring Opening Polymerization of Cyclic Esters.Dalton Trans.2019, 48, 1454–1466. Google Scholar 48. Ashida Y.; Kondo S.; Arashiba K.; Kikuchi T.; Nakajima K.; Kakimoto S.; Nishibayashi Y.A Practical Synthesis of Ammonia from Nitrogen Gas, Samarium Diiodide and Water Catalyzed by a Molybdenum–PCP Pincer Complex.Synthesis2019, 51, 3792–3795. Google Scholar 49. Bruch Q. J.; Connor G. P.; Chen C.-H.; Holland P. L.; Mayer J. M.; Hasanayn F.; Miller A. J. M.Dinitrogen Reduction to Ammonium at Rhenium Utilizing Light and Proton-Coupled Electron Transfer.J. Am. Chem. Soc.2019, 141, 20198–20208. Google Scholar 50. Kinoshita E.; Arashiba K.; Kuriyama S.; Eizawa A.; Nakajima K.; Nishibayashi Y.Synthesis and Catalytic Activity of Molybdenum–Nitride Complexes Bearing Pincer Ligands.Eur. J. Inorg. Chem.2015, 2015, 1789–1794. Google Scholar 51. Silantyev G. A.; Förster M.; Schluschaß B.; Abbenseth J.; Würtele C.; Volkmann C.; Holthausen M. C.; Schneider S.Dinitrogen Splitting Coupled to Protonation.Angew. Chem. Int. Ed.2017, 56, 5872–5876. Google Scholar 52. Laplaza C. E.; Cummins C. C.Dinitrogen Cleavage by a Three-Coordinate Molybdenum(III) Complex.Science1995, 268, 861–863. Google Scholar 53. Tsai Y.-C.; Cummins C. C.Base-Catalyzed Dinitrogen Cleavage by Molybdenum Amides.Inorg. Chim. Acta2003, 345, 63–69. Google Scholar 54. Arashiba K.; Kinoshita E.; Kuriyama S.; Eizawa A.; Nakajima K.; Tanaka H.; Yoshizawa K.; Nishibayashi Y.Catalytic Reduction of Dinitrogen to Ammonia by Use of Molybdenum-Nitride Complexes Bearing a Tridentate Triphosphine as Catalysts.J. Am. Chem. Soc.2015, 137, 5666–5669. Google Scholar Previous articleNext article FiguresReferencesRelatedDetails Issue AssignmentVolume 0Issue 0Page: 1-8Supporting Information Copyright & Permissions© 2022 Chinese Chemical SocietyKeywordsligand postmodificationnitrogen reductionPN3Psamarium(II) iodidemolybdenumAcknowledgmentsWe are grateful for the service of NOOR 2, Shaheen 2 High-Performance Computing Facilities, and financial support from King Abdullah University of Science and Technology (KAUST). We acknowledge Dr. Xingzhu Chen and Amy Perez for the graphic design. Downloaded 331 times PDF downloadLoading ...
更多查看译文
关键词
nitride complex,ammonia,ligand,phosphorus-nitrogen
AI 理解论文
溯源树
样例
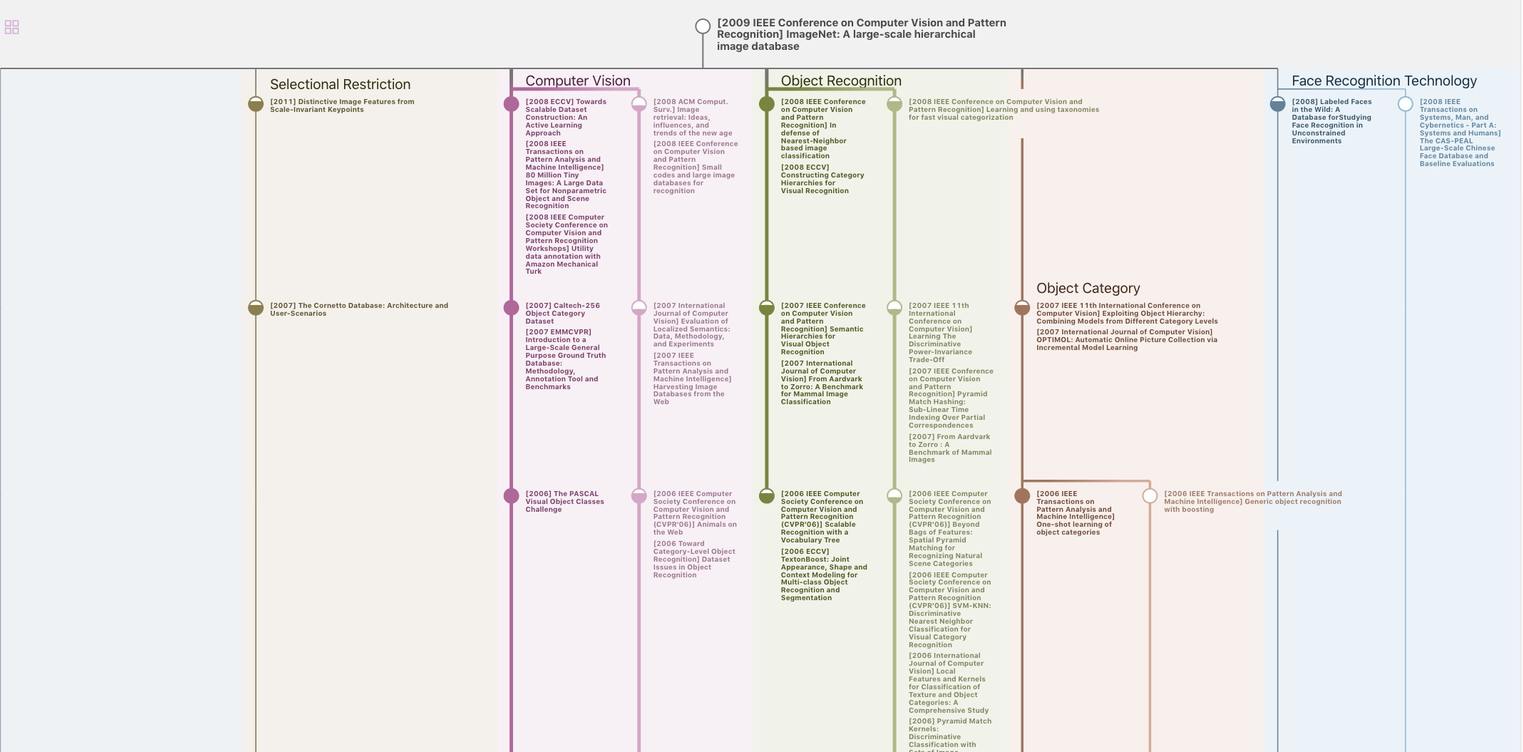
生成溯源树,研究论文发展脉络
Chat Paper
正在生成论文摘要