Noncovalent interactions that tune the reactivities of the flavins in bifurcating electron transferring flavoprotein
Journal of Biological Chemistry(2023)
摘要
Bifurcating electron transferring flavoproteins (Bf-ETFs) tune chemically identical flavins to two contrasting roles. To understand how, we used hybrid quantum mechanical molecular mechanical calculations to characterize noncovalent interactions applied to each flavin by the protein. Our computations replicated the differences between the reactivities of the flavins: the electron transferring flavin (ETflavin) was calculated to stabilize anionic semiquinone (ASQ) as needed to execute its single-electron transfers, whereas the Bf flavin (Bfflavin) was found to disfavor the ASQ state more than does free flavin and to be less susceptible to reduction. The stability of ETflavin ASQ was attributed in part to H-bond donation to the flavin O2 from a nearby His side chain, via comparison of models employing different tautomers of His. This H-bond between O2 and the ET site was uniquely strong in the ASQ state, whereas reduction of ETflavin to the anionic hydroquinone (AHQ) was associated with side chain reorientation, backbone displacement, and reorganization of its H-bond network including a Tyr from the other domain and subunit of the ETF. The Bf site was less responsive overall, but formation of the Bfflavin AHQ allowed a nearby Arg side chain to adopt an alternative rotamer that can H-bond to the Bfflavin O4. This would stabilize the anionic Bfflavin and rationalize effects of mutation at this position. Thus, our computations provide insights on states and conformations that have not been possible to characterize experimentally, offering explanations for observed residue conservation and raising possibilities that can now be tested. Bifurcating electron transferring flavoproteins (Bf-ETFs) tune chemically identical flavins to two contrasting roles. To understand how, we used hybrid quantum mechanical molecular mechanical calculations to characterize noncovalent interactions applied to each flavin by the protein. Our computations replicated the differences between the reactivities of the flavins: the electron transferring flavin (ETflavin) was calculated to stabilize anionic semiquinone (ASQ) as needed to execute its single-electron transfers, whereas the Bf flavin (Bfflavin) was found to disfavor the ASQ state more than does free flavin and to be less susceptible to reduction. The stability of ETflavin ASQ was attributed in part to H-bond donation to the flavin O2 from a nearby His side chain, via comparison of models employing different tautomers of His. This H-bond between O2 and the ET site was uniquely strong in the ASQ state, whereas reduction of ETflavin to the anionic hydroquinone (AHQ) was associated with side chain reorientation, backbone displacement, and reorganization of its H-bond network including a Tyr from the other domain and subunit of the ETF. The Bf site was less responsive overall, but formation of the Bfflavin AHQ allowed a nearby Arg side chain to adopt an alternative rotamer that can H-bond to the Bfflavin O4. This would stabilize the anionic Bfflavin and rationalize effects of mutation at this position. Thus, our computations provide insights on states and conformations that have not been possible to characterize experimentally, offering explanations for observed residue conservation and raising possibilities that can now be tested. Electron transferring flavoproteins (ETFs) have been known since the 1950s to shuttle single electron (1e) equivalents among dehydrogenases and the respiratory electron transfer chain of mitochondria and aerobic bacteria (1Beinert H. Spectral characteristics of flavins at the semiquinoid oxidation level.J. Am. Chem. Soc. 1956; 78: 5323-5328Crossref Google Scholar, 2Thorpe C. Electron-transferring flavoproteins.in: Müller F. Chemistry and Biochemistry of Flavoenzymes. CRC press, Boca Raton FL1991: 471-486Google Scholar, 3Watmough N.J. Kiss J. Frerman F.E. Structural and redox relationships between Paracoccus denitrificans, porcine and human electron-transferring flavoproteins.Eur. J. Biochem. 1992; 205: 1089-1097Crossref PubMed Scopus (18) Google Scholar). This activity is mediated by a flavin adenine dinucleotide (FAD) bound in a domain (“head” or “shuttle”) that is found in one of two orientations relative to a “base” composed of domains I and III, in crystal structures (4Toogood H.S. van Thiel A. Scrutton N.S. Leys D. Stabilization of non-productive conformations underpins rapid electron transfer to electron transferring flavoprotein.J. Biol. Chem. 2005; 280: 30361-30366Abstract Full Text Full Text PDF PubMed Scopus (32) Google Scholar). Naming of ETFs' two subunits and the domains is provided in Figure 1. In the 1970s, the first report appeared of an ETF containing a second FAD (5Whitfield C.D. Mayhew S.G. Purification and properties of electron-transferring flavoprotein from Peptostreptococcus elsdenii.J. Biol. Chem. 1974; 249: 2801-2810Abstract Full Text PDF PubMed Google Scholar), replacing what now appears to be a vestigial adenosine monophosphate (6Mohamed-Raseek N. Duan H.D. Mroginski M.A. Miller A.F. Spectroscopic, thermodynamic and computational evidence of the locations of the FADs in the nitrogen fixation-associated electron transfer flavoprotein.Chem. Sci. 2019; 10: 7762-7772Crossref PubMed Google Scholar, 7DuPlessis E.R. Rohlfs R.J. Hille R. Thorpe C. Electron-transferring flavoproteins from pig and the methylotrophic bacterium W3A1 contains AMP as well as FAD.Biochem. Mol. Biol. Int. 1994; 32: 195-199PubMed Google Scholar, 8Sato K. Nishina Y. Shiga K. Electron-transferring flavoprotein has an AMP-binding site in addition to the FAD-binding site.J. Biochem. 1993; 114: 215-222Crossref PubMed Scopus (42) Google Scholar). It has become apparent that diverse anaerobes employ such bifurcating electron transferring flavoproteins (Bf-ETFs) to generate more strongly reducing electron carriers (with lower reduction midpoint potentials, E°s) based on abundant but only modestly reducing nicotinamide adenine dinucleotide (NADH) (9Nitschke W. Russell M.J. Redox bifurcations: mechanisms and importance to life now, and at its origin.Bioessays. 2012; 34: 106-109Crossref PubMed Scopus (103) Google Scholar, 10Buckel W. Thauer R.K. Flavin-based electron bifurcation, a new mechanism of biological energy coupling.Chem. Rev. 2018; 118Crossref PubMed Scopus (183) Google Scholar). “Bifurcation,” or more accurately electron transfer bifurcation, captures excess energy released from a favorable electron transfer (ET) to higher E°, by using it to drive an unfavorable transfer (9Nitschke W. Russell M.J. Redox bifurcations: mechanisms and importance to life now, and at its origin.Bioessays. 2012; 34: 106-109Crossref PubMed Scopus (103) Google Scholar). The resulting reduced flavodoxin and ferredoxin provide reducing equivalents needed for demanding reactions including nitrogen fixation and carbon fixation (11Peters J.W. Miller A.F. Jones A.K. King P.W. Adams M.W. Electron bifurcation.Curr. Opin. Chem. Biol. 2016; 31: 146-152Crossref PubMed Scopus (93) Google Scholar, 12Buckel W. Thauer R.K. Energy conservation via electron bifurcating ferredoxin reduction and proton/Na+ translocating ferredoxin oxidation.Biochim. Biophys. Acta. 2013; 1827: 94-113Crossref PubMed Scopus (531) Google Scholar, 13Herrmann G. Jayamani E. Mai G. Buckel W. Energy conservation via electron-transferring flavoprotein in anaerobic bacteria.J. Bacteriol. 2008; 190: 784-791Crossref PubMed Scopus (301) Google Scholar). In brief, a pair of electrons is acquired from NADH by a flavin dubbed the “bifurcating flavin” (Bfflavin) because it passes each of the two electrons to separate acceptors. One electron undergoes exergonic electron transfer via the electron transferring flavin (ETflavin), thereby paying for endergonic ET of the second electron to a low-E° acceptor (Fig. 1). To prevent both of NADH's electrons from exploiting the exergonic path, it is critical that the ETflavin only accept electrons one at a time. In contrast, the Bfflavin needs to acquire electrons in pairs from NADH. Thus, it might seem surprising that nature has adopted the use of flavins for both roles. A conformational change is believed to act as a gate, separating the ETflavin from the Bfflavin after one electron has been transferred, and thereby enforcing transfer of the other electron to the other acceptor (14Demmer J.K. Bertsch J. Oppinger C. Wohlers H. Kayastha K. Demmer U. et al.Molecular basis of the flavin-based electron-bifurcating caffeyl-CoA reductase reaction.FEBS Lett. 2018; 592: 332-342Crossref PubMed Scopus (25) Google Scholar, 15Demmer J.K. Chowdhury N.P. Selmer T. Ermler U. Buckel W. The semiquinone swing in the bifurcating electron transferring flavoprotein/butyryl-coA dehydrogenase complex from Clostridium difficile.Nat. Commun. 2017; 8: 1577Crossref PubMed Scopus (67) Google Scholar). The two conformations seen in crystal structures reveal an 80° rigid-body rotation of the shuttle domain that carries the ETflavin, removing it from near the Bfflavin (16Kayastha K. Katsyv A. Himmrich C. Welsch S. Schuller J.M. Ermler U. et al.Structure-based electron-confurcation mechanism of the Ldh-EtfAB complex.eLife. 2022; 11e77095Crossref PubMed Scopus (4) Google Scholar, 17Duan H.D. Mohamed-Raseek N. Miller A.F. Spectroscopic evidence for direct flavin-flavin contact in a bifurcating electron transfer flavoprotein.J. Biol. Chem. 2020; 295: 12618-12634Abstract Full Text Full Text PDF PubMed Scopus (0) Google Scholar) to a position more than 35 Å away and interacting instead with the high-E° acceptor partner protein (4Toogood H.S. van Thiel A. Scrutton N.S. Leys D. Stabilization of non-productive conformations underpins rapid electron transfer to electron transferring flavoprotein.J. Biol. Chem. 2005; 280: 30361-30366Abstract Full Text Full Text PDF PubMed Scopus (32) Google Scholar, 14Demmer J.K. Bertsch J. Oppinger C. Wohlers H. Kayastha K. Demmer U. et al.Molecular basis of the flavin-based electron-bifurcating caffeyl-CoA reductase reaction.FEBS Lett. 2018; 592: 332-342Crossref PubMed Scopus (25) Google Scholar, 15Demmer J.K. Chowdhury N.P. Selmer T. Ermler U. Buckel W. The semiquinone swing in the bifurcating electron transferring flavoprotein/butyryl-coA dehydrogenase complex from Clostridium difficile.Nat. Commun. 2017; 8: 1577Crossref PubMed Scopus (67) Google Scholar, 18Leys D. Basran J. Talfournier F. Sutcliffe M.J. Scrutton N.S. Extensive conformational sampling in a ternary electron transfer complex.Nat. Struct. Biol. 2003; 10: 219-225Crossref PubMed Scopus (107) Google Scholar, 19Feng X. Schut G.J. Lipscomb G. Li H.Y. Adams M.W.W. Cryoelectron microscopy structure and mechanism of the membrane-associated electron-bifurcating flavoprotein Fix/EtfABCX.Proc. Natl. Acad. Sci. U. S. A. 2021; 118e2016978118Crossref Scopus (13) Google Scholar). It remains unclear how this domain movement is coupled to catalytic events and what local perturbations trigger it. However, we note that the high E° determined for reduction of the ETflavin from the oxidized state (OX) to anionic semiquinone (ASQ) (E°OX/ASQ) suggests that ETflavin will be ASQ in resting enzyme and therefore only capable of accepting one more electron. Thus, the redox tuning applied by the protein environment appears to make the ETflavin into a 1e acceptor (6Mohamed-Raseek N. Duan H.D. Mroginski M.A. Miller A.F. Spectroscopic, thermodynamic and computational evidence of the locations of the FADs in the nitrogen fixation-associated electron transfer flavoprotein.Chem. Sci. 2019; 10: 7762-7772Crossref PubMed Google Scholar, 20Duan H.D. Lubner C.E. Tokmina-Lukaszewska M. Gauss G.H. Bothner B. King P.W. et al.Distinct flavin properties underlie flavin-based electron bifurcation within a novel electron-transferring flavoprotein FixAB from Rhodopseudomonas palustris.J. Biol. Chem. 2018; 293: 4688-4701Abstract Full Text Full Text PDF PubMed Scopus (16) Google Scholar, 21Schut G.J. Mohamed-Raseek N.R. Tokmina-Lukaszewska M. Mulder D.E. Nguyen D.M.N. Lipscomb G.L. et al.The catalytic mechanism of electron bifurcating electron transfer flavoproteins (ETFs) involves an intermediary complex with NAD+.J. Biol. Chem. 2019; 294: 3271-3283Abstract Full Text Full Text PDF PubMed Scopus (27) Google Scholar). Indeed, we argue that the extreme chemical versatility of flavins has made them invaluable cofactors over the course of evolution, as different proteins can be evolved to emphasize different aspects of the flavin's repertoire by surrounding it with amino acid residues that modify the flavin electronics and accessibility in different ways (22Massey V. Hemmerich P. Active site probes of flavoproteins.Biochem. Soc. Trans. 1980; 8: 246-257Crossref PubMed Google Scholar). Thus, biosynthesis of this one cofactor enables cells to satisfy many catalytic needs (23Fagan R.L. Palfey B.A. Flavin-dependent enzymes.in: Begley T. Comprehensive Natural Products Chemistry II. Elsevier, Oxford, UK2010: 37-114Crossref Google Scholar). At issue here, the isoalloxazine core of the flavin is poised between 1e and 2e reactivity by its two 1e E°s that are weakly crossed (9Nitschke W. Russell M.J. Redox bifurcations: mechanisms and importance to life now, and at its origin.Bioessays. 2012; 34: 106-109Crossref PubMed Scopus (103) Google Scholar, 24Mayhew S.G. The effects of pH and semiquinone formation on the oxidation-reduction potentials of flavin mononucleotide: a reappraisal.Eur. J. Biochem. 1999; 265: 698-702Crossref PubMed Scopus (116) Google Scholar), resulting in predominantly 2e reduction from OX to the 2e reduced hydroquinone (HQ), but with a thermodynamically accessible semiquinone (SQ) state that can be populated to approximately 1% at neutral pH (24Mayhew S.G. The effects of pH and semiquinone formation on the oxidation-reduction potentials of flavin mononucleotide: a reappraisal.Eur. J. Biochem. 1999; 265: 698-702Crossref PubMed Scopus (116) Google Scholar). Thus, a protein site able to make relatively modest changes to the relative energies of the OX, SQ, and HQ states can shift the reactivity of the flavin between 1e and 2e transfers. Furthermore, both the SQ and HQ states have physiologically accessible pKas, of 8.5 and 6.8, respectively (24Mayhew S.G. The effects of pH and semiquinone formation on the oxidation-reduction potentials of flavin mononucleotide: a reappraisal.Eur. J. Biochem. 1999; 265: 698-702Crossref PubMed Scopus (116) Google Scholar, 25Land E.J. Swallow A.,J. One-electron reactions in biochemical systems as studied by pulse radiolysis. II. Riboflavine.Biochem. 1966; 5: 2117-2125Crossref PubMed Scopus (52) Google Scholar). Consequently, proteins can modulate local electrostatics to (dis)favor the ASQ or anionic HQ (AHQ) (26Roberts D.L. Salazar D. Fulmer J.P. Frerman F.E. Kim J.J. Crystal structure of paracoccus denitrificans electron transfer flavoprotein: structural and electrostatic analysis of a conserved flavin binding domain.Biochemistry. 1999; 38: 1977-1989Crossref PubMed Scopus (60) Google Scholar), or they can modulate the favorability of proton transfer coupled to ET. Further interventions are achieved via orchestrated changes of the local dielectric that can alter the energy associated with a given charge (27Iijima M. Ohnuki J. Sato T. Sugishima M. Takano M. Coupling of redox and structural states in cytochrome P450 reductase studied by molecular dynamics simulation.Sci. Rep. 2019; 9: 9341Crossref PubMed Scopus (9) Google Scholar). Steric interactions producing distortions characteristic of more reduced states are also expected to raise the flavin E°s (28Walsh J.D. Miller A.-F. Flavin reduction potential tuning by substitution and bending.J. Mol. Struct. (Theochem). 2003; 623: 185-195Crossref Scopus (54) Google Scholar, 29Hasford J.J. Kemnitzer W. Rizzo C.J. Conformational effects on flavin redox chemistry.J. Org. Chem. 1997; 62: 5244-5245Crossref Scopus (46) Google Scholar). In addition, the flavin π system incorporates several functionalities that engage in hydrogen bonds (H-bonds). These provide additional mechanisms by which proteins can tune the reactivity of flavins (Fig. 2). Vibrational spectroscopy reveals that the flavins' two carbonyl O atoms respond to H-bonding, and NMR reveals changes in local electron density distribution as a result of H-bonds to the O and N atoms (30Hazekawa I. Nishina Y. Sato K. Shichiri M. Miura R. Shiga K. A Raman study on the C(4)=O stretching mode of flavins in flavoenzymes: hydrogen bonding at the C(4)=O moiety.J. Biochem. 1997; 121: 1147-1154Crossref PubMed Google Scholar, 31Wille G. Ritter M. Friedemann R. Mantele W. Hubner G. Redox-triggered FTIR difference spectra of FAD in aqueous solution and bound to flavoproteins.Biochemistry. 2003; 42: 14814-14821Crossref PubMed Scopus (52) Google Scholar, 32Rüterjans H. Fleischmann G. Löhr M. Knauf F. Blümel M. Lederer F. et al.NMR studies of flavoproteins.Biochem. Soc. Trans. 1996; 24: 116-121Crossref PubMed Scopus (10) Google Scholar, 33Cui D. Koder Jr., R.L. Dutton P.L. Miller A.-F. 15N solid-state NMR as a probe of flavin H-bonding.J. Phys. Chem. -B. 2011; 115: 7788-7798Crossref PubMed Scopus (19) Google Scholar). ETFs appear to employ several of the above features to tune the relative stability of the ETflavin's ASQ (34Mohamed-Raseek N. Miller A.F. Contrasting roles for two conserved arginines: Stabilizing flavin semiquinone or quaternary structure, in bifurcating electron transfer flavoproteins.J. Biol. Chem. 2022; 298101733Abstract Full Text Full Text PDF Scopus (3) Google Scholar, 35Dwyer T.M. Zhang L. Muller M. Marrugo F. Frerman F.E. The functions of the flavin contact residues αArg249 and βTyr16, in human electron transfer flavoprotein.Biochim. Biophys. Acta. 1999; 1433: 139-152Crossref PubMed Scopus (19) Google Scholar, 36Talfournier F. Munro A.W. Basran J. Sutcliffe M.J. Daff S. Chapman S.K. et al.Alpha Arg-237 in Methylophilus methylotrophus (sp. W3A1) electron-transferring flavoprotein affords approximately 200-millivolt stabilization of the FAD anionic semiquinone and a kinetic block on full reduction to the dihydroquinone.J. Biol. Chem. 2001; 276: 20190-20196Abstract Full Text Full Text PDF PubMed Scopus (32) Google Scholar). A positively charged Arg or Lys adjacent to the ETflavin is conserved in 98% of Bf-ETFs and has been shown to stabilize the ASQ and impede formation of the AHQ (34Mohamed-Raseek N. Miller A.F. Contrasting roles for two conserved arginines: Stabilizing flavin semiquinone or quaternary structure, in bifurcating electron transfer flavoproteins.J. Biol. Chem. 2022; 298101733Abstract Full Text Full Text PDF Scopus (3) Google Scholar, 35Dwyer T.M. Zhang L. Muller M. Marrugo F. Frerman F.E. The functions of the flavin contact residues αArg249 and βTyr16, in human electron transfer flavoprotein.Biochim. Biophys. Acta. 1999; 1433: 139-152Crossref PubMed Scopus (19) Google Scholar, 36Talfournier F. Munro A.W. Basran J. Sutcliffe M.J. Daff S. Chapman S.K. et al.Alpha Arg-237 in Methylophilus methylotrophus (sp. W3A1) electron-transferring flavoprotein affords approximately 200-millivolt stabilization of the FAD anionic semiquinone and a kinetic block on full reduction to the dihydroquinone.J. Biol. Chem. 2001; 276: 20190-20196Abstract Full Text Full Text PDF PubMed Scopus (32) Google Scholar, 37Yang K.Y. Swenson R.P. Modulation of the redox properties of the flavin cofactor through hydrogen-bonding interactions with the N(5) atom: role of alpha Ser254 in the electron-transfer flavoprotein from the methylotrophic bacterium W3A1.Biochem. 2007; 46: 2289-2297Crossref PubMed Scopus (25) Google Scholar). Bf-ETFs also conserve a Thr or a Ser near the N5 of the ETflavin and substitution of this residue affects the E°s (37Yang K.Y. Swenson R.P. Modulation of the redox properties of the flavin cofactor through hydrogen-bonding interactions with the N(5) atom: role of alpha Ser254 in the electron-transfer flavoprotein from the methylotrophic bacterium W3A1.Biochem. 2007; 46: 2289-2297Crossref PubMed Scopus (25) Google Scholar). In addition, a His near ETflavin's O2 is conserved in 90% of Bf-ETFs but has not been as well characterized in the literature (statistics are based on an alignment of 228 Bf-ETF sequences (38Garcia Costas A.M. Poudel S. Miller A.-F. J S.G. Ledbetter R.N. Fixen K. et al.Defining electron bifurcation in the electron transferring flavoprotein family.J. Bacteriol. 2017; 199e00440-17Crossref PubMed Scopus (46) Google Scholar)). The Bf site also possesses a 99% conserved Arg (otherwise Lys). Unfortunately, its substitution destabilizes the protein and abrogates flavin binding. Therefore, little is known about how it may affect the reactivity of the flavin (34Mohamed-Raseek N. Miller A.F. Contrasting roles for two conserved arginines: Stabilizing flavin semiquinone or quaternary structure, in bifurcating electron transfer flavoproteins.J. Biol. Chem. 2022; 298101733Abstract Full Text Full Text PDF Scopus (3) Google Scholar). Moreover, because the ASQ state of Bfflavin does not normally accumulate in high-quality ETF preparations, it is not possible to study it experimentally to learn how the protein destabilizes it. Thus, computational approaches can fill a critical knowledge gap, elucidating mechanisms of tuning applicable to the Bfflavin, while using the more accessible ETflavin as a control for which computations can be validated by experiments (6Mohamed-Raseek N. Duan H.D. Mroginski M.A. Miller A.F. Spectroscopic, thermodynamic and computational evidence of the locations of the FADs in the nitrogen fixation-associated electron transfer flavoprotein.Chem. Sci. 2019; 10: 7762-7772Crossref PubMed Google Scholar). We begin by validating our computational approach (see "Validation: Computational Replication of Contrasting Flavin Reactivities"), then provide a comparative overview of interactions observed in each of the two sites (see "Overview of the Sites' Geometries and Protein–Flavin Interactions"), before describing striking electrostatic differences between them, in the section on "Effect of Protein Electrostatics on Distribution of Electron Density in the Flavins." In the section "Interactions in the ET site," examination of H-bonding in the ET site identifies the interplay between H-bonding at ETO2, formation of ETASQ, and the protonation state of ETN5, which enables a conserved His at position 290 to influence reactivity at ETN5. Although the crystallographic Bf site seems considerably less responsive to flavin oxidation state than the ET site, based on our models obtained by energy minimization (see "The Bf Site: H-Bonding at BfN5") a model incorporating an alternative rotamer of R146 raises interesting new possibilities (see "H-Bonding by Another Rotamer of R146, for the AHQ of BfFlavin") and suggests a basis for the high conservation of this residue. Before using our models to explain the contrasting reactivities of the two flavins, we tested whether they succeed in reproducing the differences. In experiments, the ET site stabilizes ETASQ, populating this state to 90% during stepwise reductions (39Sucharitakul J. Buttranon S. Wongnate T. Chowdhury N.P. Prongjit M. Buckel W. et al.Modulations of the reduction potentials of flavin-based electron bifurcation complexes and semiquinone stabilities are key to control directional electron flow.FEBS J. 2020; https://doi.org/10.1111/febs.15343Crossref PubMed Scopus (14) Google Scholar). In contrast, free flavin only accumulates 1% of the population as ASQ, whereas ≥99% undergoes 2e reduction directly from OX to HQ (24Mayhew S.G. The effects of pH and semiquinone formation on the oxidation-reduction potentials of flavin mononucleotide: a reappraisal.Eur. J. Biochem. 1999; 265: 698-702Crossref PubMed Scopus (116) Google Scholar). Thus, a favorable ASQ state, specifically in the ET site, is a critical test of whether our models correctly describe this site. The ET site also makes bound flavin more amenable to reduction than free flavin, whereas the Bf site has the opposite effect (6Mohamed-Raseek N. Duan H.D. Mroginski M.A. Miller A.F. Spectroscopic, thermodynamic and computational evidence of the locations of the FADs in the nitrogen fixation-associated electron transfer flavoprotein.Chem. Sci. 2019; 10: 7762-7772Crossref PubMed Google Scholar, 20Duan H.D. Lubner C.E. Tokmina-Lukaszewska M. Gauss G.H. Bothner B. King P.W. et al.Distinct flavin properties underlie flavin-based electron bifurcation within a novel electron-transferring flavoprotein FixAB from Rhodopseudomonas palustris.J. Biol. Chem. 2018; 293: 4688-4701Abstract Full Text Full Text PDF PubMed Scopus (16) Google Scholar, 21Schut G.J. Mohamed-Raseek N.R. Tokmina-Lukaszewska M. Mulder D.E. Nguyen D.M.N. Lipscomb G.L. et al.The catalytic mechanism of electron bifurcating electron transfer flavoproteins (ETFs) involves an intermediary complex with NAD+.J. Biol. Chem. 2019; 294: 3271-3283Abstract Full Text Full Text PDF PubMed Scopus (27) Google Scholar, 39Sucharitakul J. Buttranon S. Wongnate T. Chowdhury N.P. Prongjit M. Buckel W. et al.Modulations of the reduction potentials of flavin-based electron bifurcation complexes and semiquinone stabilities are key to control directional electron flow.FEBS J. 2020; https://doi.org/10.1111/febs.15343Crossref PubMed Scopus (14) Google Scholar), so a test of our Bf site models is that they should disfavor flavin reduction. Our models succeed on both criteria. Based on Gibbs free energy differences (Table 1), formation of ASQ from OX is favorable in the ET site, only. The contrasting computed energy differences clearly stem from the protein, as the two sites produced deviations in opposing directions from the value for gas-phase flavin. Second, formation of AHQ was calculated to be less favorable in the Bf site than in either the ET site or the gas phase, validating our models of that site as well. Although qualitative, these successes are impressive considering the many aspects of protein environments that can contribute to flavin reactivity (see the introduction section), in conjunction with the computational parsimony required to permit the desired characterizations of the numerous states believed to contribute to reactivity.Table 1Gibbs free energy differences between flavin oxidation states in each of the binding sites of AfeETFaFor the protein-bound flavins, the protein environment was represented as a distribution of electrostatic point charges. Comparisons can be made only within individual columns. The large difference between the sets of energies associated with each of the two chemical events may reflect our neglect of intrinsic energies of the electrons and proton that feature in the balanced equations for the different reductions.,bFor completeness, corresponding electronic energy differences are given in Table S1.Flavin’s environmentΔG [kcal/mol]ASQ - OXAHQ - OXGas phase LF−36−408Bfflavin4−352ETflavinδ−73−385ETflavinε−73−388a For the protein-bound flavins, the protein environment was represented as a distribution of electrostatic point charges. Comparisons can be made only within individual columns. The large difference between the sets of energies associated with each of the two chemical events may reflect our neglect of intrinsic energies of the electrons and proton that feature in the balanced equations for the different reductions.b For completeness, corresponding electronic energy differences are given in Table S1. Open table in a new tab Because they replicate the different reactivities of the two flavins, our computations provide a basis for evaluating the roles played by different aspects of the protein environment. Here we have considered local electrostatics, geometry imposed by the protein, and H-bonds. Such factorization is difficult in experiments, where residue substitutions tend to change all three contributions at once. Our computations reveal interplay between each flavin and protein residues nearby, as summarized in Figure 3. H-bonding with flavin positions N3 and O4 was found to be mainly with protein backbone carbonyl (=O) and amide (NH) groups and therefore could be coupled to higher-order structure. However H-bonds to flavin O2 were also provided by side chains or a water molecule, which can be more mobile. H-bond donation to flavin N5 was also via amino acid side chains in both sites, which is significant because the 5 position is converted from an H-bond acceptor to an H-bond donor upon acquisition of a proton as part of reduction to form AHQ (Fig. 2). Thus, ETF's presentation of side chains to that position obviates a need for the backbone reorientation seen in flavodoxin (40Ludwig M.L. Pattridge K.A. Metzger A.L. Dixon M.M. Eren M. Feng Y. et al.Control of oxidation-reduction potentials in flavodoxin from Clostridium beijerinckii: the role of conformational changes.Biochemistry. 1997; 36: 1259-1280Crossref PubMed Scopus (159) Google Scholar). In both sites, reorientation of the side chain interacting at N5 enabled the protein to accept an H-bond from the flavin AHQ, where it had donated one to OX and ASQ (see sections on "Interactions in the ET site" and "The Bf site: H-bonding at BfN5"). Reduction of ETflavin to ETAHQ caused the side chains of S270 and Q266 to reorient, reversing the polarities of their interactions with the ETflavin. In addition, a segment of backbone at G271 relinquished its H-bond to ETO4 and moved away by 1 Å (Fig. 4A). Thus, the protein constituents of our computed ET site are conformationally responsive to the oxidation state of ETflavin and not frozen in the ETOX crystal structure. Our computations thus suggest that the ET site supports facile ETflavin reduction via provision of a highly fluid or adaptive network of H-bonds from mobile side chains and backbone loops. Electrostatic stabilization of the different flavin oxidation states by each of the protein sites was assessed
更多查看译文
关键词
conformational change,electron density,electron transfer,electron transfer flavoprotein,electron bifurcation,flavoprotein,FAD,hydrogen bond,QM/MM,redox tuning
AI 理解论文
溯源树
样例
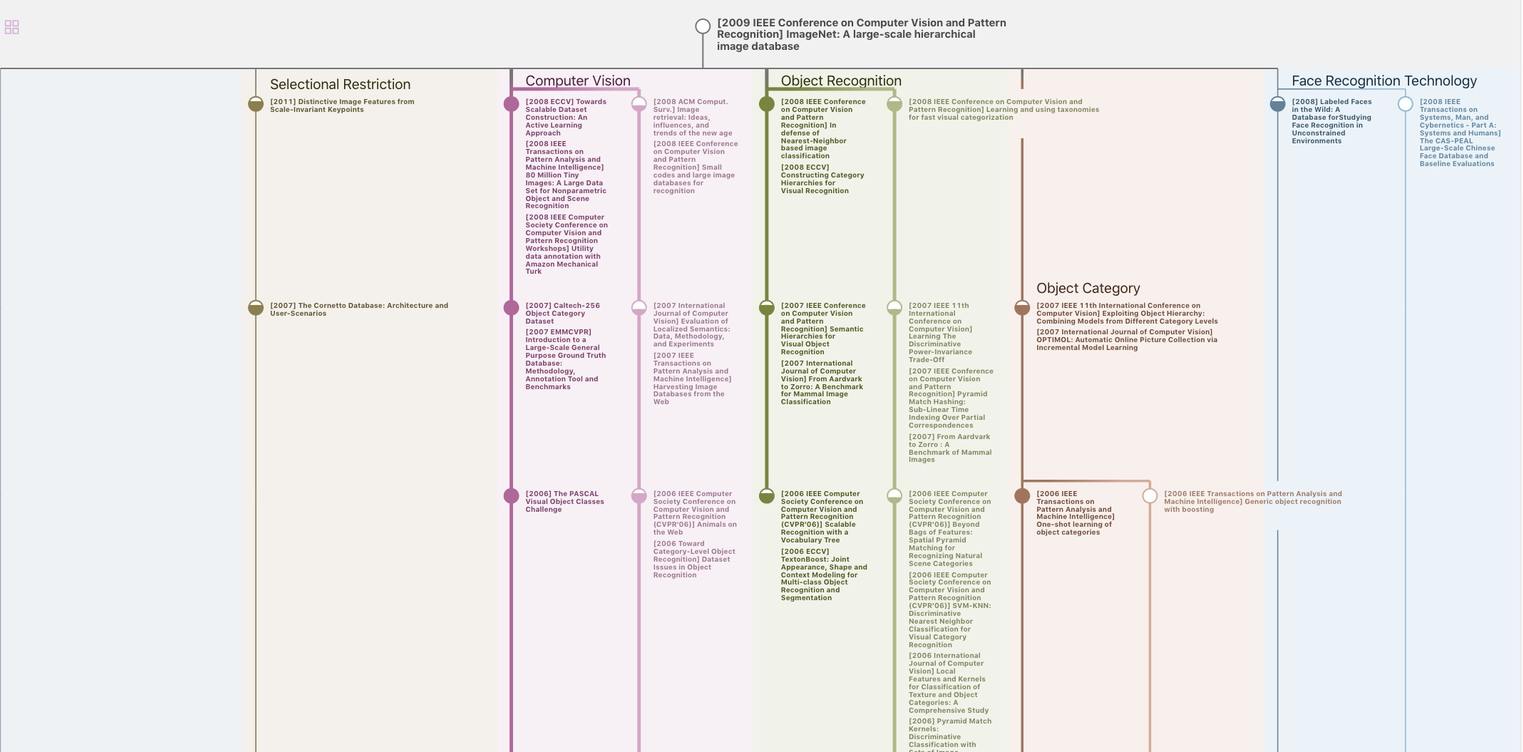
生成溯源树,研究论文发展脉络
Chat Paper
正在生成论文摘要