Author response: A widely distributed metalloenzyme class enables gut microbial metabolism of host- and diet-derived catechols
crossref(2019)
摘要
Article Figures and data Abstract eLife digest Introduction Results Discussion Materials and methods Appendix 1 Data availability References Decision letter Author response Article and author information Metrics Abstract Catechol dehydroxylation is a central chemical transformation in the gut microbial metabolism of plant- and host-derived small molecules. However, the molecular basis for this transformation and its distribution among gut microorganisms are poorly understood. Here, we characterize a molybdenum-dependent enzyme from the human gut bacterium Eggerthella lenta that dehydroxylates catecholamine neurotransmitters. Our findings suggest that this activity enables E. lenta to use dopamine as an electron acceptor. We also identify candidate dehydroxylases that metabolize additional host- and plant-derived catechols. These dehydroxylases belong to a distinct group of largely uncharacterized molybdenum-dependent enzymes that likely mediate primary and secondary metabolism in multiple environments. Finally, we observe catechol dehydroxylation in the gut microbiotas of diverse mammals, confirming the presence of this chemistry in habitats beyond the human gut. These results suggest that the chemical strategies that mediate metabolism and interactions in the human gut are relevant to a broad range of species and habitats. eLife digest Inside the human gut there are trillions of bacteria. These microbes are critical for breaking down and modifying molecules that the body consumes (such as nutrients and drugs) and produces (such as hormones). Although metabolizing these molecules is known to impact health and disease, little is known about the specific components, such as the genes and enzymes, involved in these reactions. A prominent microbial reaction in the gut metabolizes molecules by removing a hydroxyl group from an aromatic ring and replacing it with a hydrogen atom. This chemical reaction influences the fate of dietary compounds, clinically used drugs and chemicals which transmit signals between nerves (neurotransmitters). But even though this reaction was discovered over 50 years ago, it remained unknown which microbial enzymes are directly responsible for this metabolism. In 2019, researchers discovered the human gut bacteria Eggerthella lenta produces an enzyme named Dadh that can remove a hydroxyl group from the neurotransmitter dopamine. Now, Maini Rekdal et al. – including many of the researchers involved in the 2019 study – have used a range of different experiments to further characterize this enzyme and see if it can break down molecules other than dopamine. This revealed that Dadh specifically degrades dopamine, and this process promotes E. lenta growth. Next, Maini Rekdal et al. uncovered a group of enzymes that had similar characteristics to Dadh and could metabolize molecules other than dopamine, including molecules derived from plants and nutrients in food. These Dadh-like enzymes were found not only in the guts of humans, but in other organisms and environments, including the soil, ocean and plants. Plant-derived molecules are associated with human health, and the discovery of the enzymes that break down these products could provide new insights into the health effects of plant-based foods. In addition, the finding that gut bacteria harbor a dopamine metabolizing enzyme has implications for the interaction between the gut microbiome and the nervous system, which has been linked to human health and disease. These newly discovered enzymes are also involved in metabolic reactions outside the human body. Future work investigating the mechanisms and outputs of these reactions could improve current strategies for degrading pollutants and producing medically useful molecules. Introduction The human gastrointestinal tract is one of the densest microbial habitats on Earth. Possessing 150-fold more genes than the human genome, the trillions of organisms that make up this community (the human gut microbiota) harbor metabolic capabilities that expand the range of chemistry taking place in the body (Koppel et al., 2017; Qin et al., 2010; Sender et al., 2016). Microbial metabolism affects host nutrition and health by breaking down otherwise inaccessible carbohydrates, biosynthesizing essential vitamins, and transforming endogenous and exogenous small molecules into bioactive metabolites (Koppel and Balskus, 2016). Gut microbial activities can also vary significantly between individuals, affecting the toxicity and efficacy of drugs (Zimmermann et al., 2019; Koppel et al., 2018; Gopalakrishnan et al., 2018; Wallace et al., 2010; Haiser et al., 2013), susceptibility to infection (Buffie et al., 2015; Devlin and Fischbach, 2015), and host metabolism (Yao et al., 2018; Romano et al., 2017). To decipher the biological roles of gut microbial metabolism, it is critical that we uncover the enzymes responsible for prominent transformations. This will not only increase the information gained from microbiome sequencing data but may also illuminate strategies for manipulating and studying microbial functions. Yet, the vast majority of gut microbial metabolic reactions have not yet been linked to specific enzymes. A prominent but poorly understood gut microbial activity is the dehydroxylation of catechols (1,2-dihydroxylated aromatic rings), a structural motif commonly found in a diverse range of compounds that includes dietary phytochemicals, host neurotransmitters, clinically used drugs, and microbial siderophores (Wilson et al., 2016; Ozdal et al., 2016; Yang et al., 2007) (Figure 1A). Discovered over six decades ago, catechol dehydroxylation is a uniquely microbial reaction that selectively replaces the para hydroxyl group of the catechol with a hydrogen atom (Scheline et al., 1960) (Figure 1A). This reaction is particularly challenging due to the stability of the aromatic ring system. Prominent substrates for microbial dehydroxylation include the drug fostamatinib (Sweeny et al., 2010), the catecholamine neurotransmitters norepinephrine and dopamine (Smith et al., 1964; Sandler et al., 1971), the phytochemicals ellagic acid (found in nuts and berries), caffeic acid (a universal lignin precursor in plants), and catechin (present in chocolate and tea) (Peppercorn and Goldman, 1972; Cerdá et al., 2005; Takagaki and Nanjo, 2010) (Figure 1B). Dehydroxylation alters the bioactivity of the catechol compound (Kim et al., 2016; Ryu et al., 2016) and produces metabolites that act both locally in the gut and systemically to influence human health and disease (Sweeny et al., 2010; Ryu et al., 2016; Kang et al., 2016; Pietinen et al., 2001; Mabrok et al., 2012; Maini Rekdal et al., 2019; Singh et al., 2019). However, the gut microbial enzymes responsible for catechol dehydroxylation have remained largely unknown. We recently reported the discovery of a catechol dehydroxylating enzyme from the prevalent human gut Actinobacterium Eggerthella lenta. This enzyme participates in an interspecies gut microbial pathway that degrades the Parkinson’s disease medication L-dopa by catalyzing the regioselective p-dehydroxylation of dopamine to m-tyramine (Maini Rekdal et al., 2019). To identify the enzyme, we grew E. lenta strain A2 with and without dopamine and used RNA sequencing (RNA-seq) to find genes induced by dopamine. Only 15 genes were significantly upregulated in the presence of dopamine, including a putative molybdenum-dependent enzyme that was induced >2500 fold. Hypothesizing this gene encoded the dopamine dehydroxylase, we purified the enzyme from E. lenta and confirmed its activity in vitro. Dopamine dehydroxylase (Dadh) is predicted to bind bis-molybdopterin guanine nucleotide (bis-MGD), a complex metallocofactor that contains a catalytically essential molybdenum atom (Hille et al., 2014). Our previous work illuminated a role for Dadh in dopamine metabolism by pure strains and complex communities. Here, we sought to explore the substrate scope of Dadh and its broader role in catechol dehydroxylation by the gut microbiota. Results A molybdenum-dependent enzyme from Eggerthella lenta specifically metabolizes catecholamines that are available in the gut Because the human gut microbiota metabolizes a range of catecholic compounds (Figure 1B), we first investigated whether the recently discovered Dadh possessed promiscuous dehydroxylase activity. We evaluated the reactivity of natively purified E. lenta A2 Dadh towards a panel of established or potential host- and diet-derived catechol substrates (Supplementary file 1a and Figure 1—figure supplement 1). This enzyme displayed a narrow substrate scope, metabolizing only dopamine and the structurally related neurotransmitter norepinephrine, which differ only by the presence of a benzylic hydroxyl group (Figure 1C). To identify the elements necessary for substrate recognition by Dadh, we profiled its activity towards synthetic and commercially available dopamine analogs (Figure 1D, Figure 1—figure supplement 1, and Supplementary file 1b). We found that Dadh tolerated only minor modifications to the dopamine scaffold, including a single N-methylation and the presence of additional hydroxyl groups on the aromatic ring (Figure 1E). The catechol moiety was absolutely necessary for activity, and dehydroxylation required that at least one hydroxyl group be in the para position relative to the aminoethyl substituent. These data demonstrated that Dadh specifically recognizes the catecholamine scaffold. Figure 1 with 2 supplements see all Download asset Open asset An enzyme from the prevalent human gut Actinobacterium Eggerthella lenta specifically metabolizes catecholamines that are available in the gut. (A) The catechol structural motif is dehydroxylated by the gut microbiota. (B) Examples of catechols known to be dehydroxylated by gut microbes. Red indicates the carbon-oxygen bond that is broken or the hydroxyl group that is removed in the dehydroxylation reaction. (C) Activity of natively purified Dadh towards a panel of physiologically relevant catechol substrates. Enzyme (0.1 µM) was incubated with substrate (500 µM) for 22 hr at room temperature, followed by analysis using LC-MS. Bars represent the mean ±the standard error (SEM) of three biological replicates (enzyme reactions). This experiment was repeated three times. See Supplementary file 1a for the full chemical structures. (D) Dopamine analogs evaluated in this study. (E) Activity of natively purified Dadh towards dopamine analogs in C). Enzyme (0.1 µM) was incubated with substrate (500 µM) for 22 hr at room temperature, followed by analysis using LC-MS. Bars represent the mean ±the SEM of three biological replicates (enzyme reactions). See Supplementary file 1b for the full chemical structures. This experiment was performed three times. (F) Transcriptional induction and whole-cell dehydroxylation activity of E. lenta A2 in response to dopamine and a subset of dopamine analogs (500 µM each). Transcriptional induction was assessed using RNA-seq, with the fold induction shown on the x-axis (foldchange >2, FDR < 0.01). To assess whole-cell metabolism, E. lenta was grown anaerobically for 48 hr in BHI medium with 500 µM of each substrate, and the culture supernatant was analyzed for dehydroxylated metabolites using LC-MS. RNA-sequencing data represent the log2fold change from n = 3 independent cultures for each condition (compound/vehicle). The metabolism data represent the mean ±the SEM of three biological replicates (independent bacterial cultures). The culturing and analysis of metabolism was performed twice, while RNA-sequencing was done once. All raw data from Figure 1 can be found in Figure 1—source data 1. Figure 1—source data 1 Data from Dadh enzyme reactions and from studies of dadh regulation (Figure 1). https://cdn.elifesciences.org/articles/50845/elife-50845-fig1-data1-v1.xlsx Download elife-50845-fig1-data1-v1.xlsx This result prompted us to explore whether the transcriptional regulation of Dadh displayed similar specificity. Thus, we cultured E. lenta A2 in the presence of a subset of the dopamine analogs that we had tested in the previous experiment, measured dehydroxylation using liquid chromatography-mass spectrometry (LC-MS), and profiled the global transcriptome using RNA-seq. We found that the regulation of dadh was also specific for the catecholamine scaffold (Figure 1F, Supplementary file 1c). While the catecholamines dopamine and norepinephrine induced dadh expression and were dehydroxylated by E. lenta, analogs lacking the catechol (analog 1 in Figure 1D) or having a shorter side chain (analog 9 in Figure 1D) did not induce a transcriptional or metabolic response (Figure 1F, Supplementary file 1c) (Maini Rekdal et al., 2019). Together with our biochemical results, these transcriptional data suggest that Dadh may have evolved for the purpose of catecholamine neurotransmitter metabolism in E. lenta. We propose that dopamine is an endogenous substrate of this enzyme, because it was the best substrate both in vitro and in vivo, induced the highest levels of expression in E. lenta, and is produced at substantial levels within the human gastrointestinal tract (Eisenhofer et al., 1997). In addition to uncovering a preference for the catecholamine scaffold, the substrate scope of Dadh reveals potential mechanistic distinctions between this enzyme and the only other biochemically characterized reductive aromatic dehydroxylase, 4-hydroxybenzoyl Coenzyme A (CoA) reductase (4-HCBR) (Unciuleac et al., 2004). 4-HCBR is a distinct molybdenum dependent-enzyme containing a monomeric molybdopterin co-factor that uses a Birch reduction-like mechanism to remove a single aromatic hydroxyl group from 4-hydroxybenzoyl CoA. While 4-HCBR requires an electron-withdrawing thioester group to stabilize radical anion intermediates (Unciuleac et al., 2004), Dadh does not require an electron-withdrawing substituent and can tolerate additional electron-donating hydroxyl groups (Figure 1D and E, analogs 11–13). We preliminarily propose a mechanism for Dadh in which the dopamine p-hydroxyl group coordinates to the molybdenum center. This could be followed by tautomerization of the m-hydroxyl group to a ketone with protonation of the adjacent carbon atom. Oxygen atom transfer to molybdenum could be accompanied by rearomatization, providing the dehydroxylated product (Figure 1—figure supplement 2). Our proposal is consistent with the postulated mechanisms of other oxygen transfer reactions catalyzed by bis-MGD enzymes (Tenbrink et al., 2011; Hille et al., 2014). Dopamine promotes gut bacterial growth by serving as an alternative electron acceptor The specificity of Dadh for dopamine suggested this metabolic activity might have an important physiological role in E. lenta. We noted the chemical parallels between catechol dehydroxylation and reductive dehalogenation, a metabolic process in which halogenated aromatics serve as alternative electron acceptors in certain environmental bacteria (Holliger et al., 1998). This insight inspired the hypothesis that dopamine dehydroxylation could serve a similar role in gut bacteria. While we observed no growth benefit when E. lenta was grown in complex BHI medium containing dopamine (Figure 2—figure supplement 1), we found that including dopamine in a minimal medium lacking electron acceptors (basal medium) increased the endpoint optical density of E. lenta cultures (Figure 2A). This growth-promoting effect was only observed in dopamine-metabolizing E. lenta strains, as non-metabolizing strains that express an apparently inactive enzyme (Maini Rekdal et al., 2019) did not gain a growth advantage (Figure 2A and Figure 2—figure supplement 2). The effect of dopamine on E. lenta contrasts with recent studies of digoxin, a drug that is reduced by E. lenta without impacting growth in the same medium (Koppel et al., 2018). Figure 2 with 7 supplements see all Download asset Open asset Dopamine increases gut bacterial growth by serving as an alternative electron acceptor. (A) Growth of dopamine metabolizing and non-metabolizing E. lenta strains in minimal medium limited in electron acceptors (basal medium) containing 10 mM acetate. Strains were grown anaerobically for 48–72 hr at 37°C before growth was assessed. Bars represent the mean ±the SEM of three biological replicates (bacterial cultures). The experiment was performed once. (B) Tungstate inhibits of growth and dopamine metabolism by E. lenta A2 in basal medium containing 10 mM acetate. E. lenta was grown anaerobically for 48 hr at 37°C. Dopamine, m-tyramine, and nitrate were added to a final concentration of 1 mM, while DMSO was added to a final concentration of 14 mM at the time of inoculation. Tungstate (WO42–) and molybdate (MoO42–) were added to a final concentration of 0.5 mM. Bars represent the mean ±the SEM of three biological replicates (bacterial cultures). The experiment was performed twice. (C) Competition of dopamine metabolizing (Valencia) and non-metabolizing (W1BHI6) E. lenta strains in basal medium containing 10 mM acetate. Strains were grown together for 72 hr at 37°C and were then plated on BHI medium. Antibiotic resistance was used to determine strain identity. Bars represent the mean ±the SEM of six biological replicates (bacterial cultures). (***p=0.0007, two-tailed unpaired t-test). The experiment was performed twice. (D) Growth of defined gut bacterial consortia containing dopamine metabolizing and non-metabolizing E. lenta strains in basal medium containing 10 mM acetate. Tetracycline resistant (TetR) E. lenta strains were grown with tetracycline sensitive (TetS) gut isolates for 48 hr at 37°C. Plating on BHI medium containing tetracycline allowed enumeration of E. lenta. Bars represent the mean ±the SEM of three biological replicates (bacterial cultures). The experiment was performed twice. (E) Abundance of dadh in complex human gut communities cultured ex vivo. Samples from unrelated individuals (n = 24) were grown for 72 hr at 37°C in basal medium containing 10 mM acetate with or without dopamine and qPCR was used to assess abundance of dadh. Two individuals were excluded from this analysis as they did not demonstrate quantitative metabolism of dopamine after incubation. Each point represents a different individual. Lines connect data from the same individual between the two conditions. (***p=0.0005, two-tailed unpaired t-test, n = 22 samples per group). The experiment was performed twice. (F) Counts of dadh variants in the presence and absence of dopamine. The same gDNA used in E) was used to amplify full-length dadh and determine the SNP status at position 506 using Sanger sequencing. As in panel E, two individuals were removed prior to analysis as they did not demonstrate quantitative metabolism of dopamine after incubation. In addition, one individual was not included in this analysis due to failure of obtaining high quality sequencing data. (**p=0.008, Fisher’s exact test, n = 9 CGC samples and n = 12 CGC/AGC samples for vehicle; n = 18 CGC samples and n = 3 CGC/AGC samples for dopamine). The sequencing was performed once. All data , and details of the statistical tests, can be found in Figure 2—source data 1. Figure 2—source data 1 Growth data from studies of impact of dopamine on E. lenta growth in basal medium lacking electron acceptors (Figure 2). https://cdn.elifesciences.org/articles/50845/elife-50845-fig2-data1-v1.xlsx Download elife-50845-fig2-data1-v1.xlsx We further investigated the relationship between dopamine and bacterial growth in the metabolizing strain E. lenta A2. The growth increase observed in response to dopamine was dose-dependent (Figure 2—figure supplement 3), mirrored the effects of the known electron acceptors DMSO and nitrate (Koppel et al., 2018; Sperry and Wilkins, 1976), and did not derive from the product of dopamine dehydroxylation, m-tyramine (Figure 2B). Additionally, the growth benefit was directly tied to dopamine dehydroxylation. Inclusion of tungstate in the growth medium, which inactivates the big-MGD cofactor of Dadh, blocked metabolism and inhibited the growth increase. In contrast, inclusion of molybdate in the growth medium did not impact growth or metabolism (Figure 2B and Figure 2—figure supplement 4). Molybdate and tungstate alone did not impact E. lenta A2 growth in the basal medium (Figure 2—figure supplement 5). Taken together, these results indicate that active metabolism of dopamine provides a growth advantage to E. lenta, likely by serving as an alternative electron acceptor. We next examined whether dopamine could promote E. lenta growth in microbial communities. First, we competed dopamine metabolizing and non-metabolizing E. lenta strains in minimal medium. E. lenta is genetically intractable, preventing the use of engineered plasmids encoding defined fluorescence or antibiotic resistance as markers of strain identity. Instead, we took advantage of intrinsic differences in tetracyline (Tet) resistance to differentiate the closely related strains in pairwise competitions (Bisanz et al., 2018). Inclusion of dopamine in growth medium significantly increased the proportion of the metabolizer relatively to the non-metabolizer in this competition experiment (p<0.001, two-tailed unpaired t-test) (Figure 2C and Figure 2—figure supplement 6). This was driven by the growth increase of the metabolizer rather than a decrease in the non-metabolizer (Figure 2C and Figure 2—figure supplement 6). Next, we explored the impact of dopamine on Tet-resistant E. lenta in the presence of a defined bacterial community representing the major phylogenetic diversity in the human gut (Figure 2D and Supplementary file 1d) (Devlin et al., 2016; Romano et al., 2015). We found that including dopamine in the medium boosted the growth of metabolizers by an order of magnitude while non-metabolizing strains did not gain a growth advantage (Figure 2D). Finally, we evaluated the impact of dopamine on E. lenta strains present in complex human gut microbiotas. We cultured fecal samples from 24 unrelated subjects ex vivo in the presence and absence of dopamine and used qPCR to assess the abundance of E. lenta and dadh. We found that both dadh and E. lenta significantly increased by an order of magnitude in cultures containing dopamine (p<0.005, two-tailed unpaired t-test) (Figure 2E) (Figure 2—figure supplement 7). Finally, we amplified the full length dadh gene from these cultures and sequenced the region harboring the SNP that distinguishes metabolizing and non-metabolizing strains (Maini Rekdal et al., 2019). These assays indicated that the increase in dadh abundance in the complex communities was accompanied by a shift from a mixture of inactive and active dadh variants to a dominance of the metabolizing R506 variant (p<0.01, Fisher’s exact test) (Figure 2F). Finally, we noticed in these growth assays that a small number of samples did not display an increase in E. lenta or dadh abundance (n = 4 and n = 3 samples, respectively) (Figure 2E and Figure 2—figure supplement 7). While the factors influencing this outcome are unclear, they could include the possibility that these specific communities support the growth of E. lenta in other ways that such that dopamine metabolism does not provide any additional advantage, that these samples contain inhibitory factors, or that organisms not targeted by our primers were responsible for metabolism. Altogether, these results are consistent with the hypothesis that dopamine dehydroxylation can increase the fitness of metabolizing E. lenta strains in microbial communities. A screen of human gut Actinobacteria uncovers dehydroxylation of host- and plant-derived catechols Having uncovered Dadh’s specialized role in gut bacterial dopamine metabolism, we sought to identify additional gut bacterial strains and enzymes that could dehydroxylate other catechol substrates. Among human gut bacteria, only Eggerthella and closely related members of the Actinobacteria phylum have been reported to perform catechol dehydroxylation. For example, Eggerthella metabolizes dopamine (Maini Rekdal et al., 2019) and (+)-catechin (Takagaki and Nanjo, 2015), while related Gordonibacter species dehydroxylate ellagic acid (Selma et al., 2014) and didemethylsecoisolariciresinol (dmSECO), an intermediate in the multi-step biosynthesis of the anti-cancer metabolite enterodiol (Bess et al., 2020). These reports suggest that Actinobacteria could be a promising starting point to identify new dehydroxylating strains and enzymes. Thus, we screened a library of related gut Actinobacteria (Bisanz et al., 2018) (n = 3 replicates for each strain) for metabolism of a range of compounds relevant in the human gut, including plant- and host-derived small molecules, bacterial siderophores, and FDA-approved catecholic drugs (Wilson et al., 2016; Ozdal et al., 2016; Yang et al., 2007) (Supplementary file 1e) (Figure 3A). We initially used a colorimetric assay that detects catechols to assess metabolism, which allowed us to rapidly screen for potential catechol depletion across the collection of 25 strains. Figure 3 with 1 supplement see all Download asset Open asset Dehydroxylation of DOPAC, (+)-catechin, and hydrocaffeic acid by Gordonibacter pamelaeae 3C and Eggerthella lenta A2. (A-C) Pathways for metabolism of (A) DOPAC, (B) (+)-catechin, (C) and hydrocaffeic acid by human gut Actinobacteria. While DOPAC and hydrocaffeic acid are dehydroxylated directly, (+)-catechin metabolism proceeds by initial benzyl ether reduction followed by dehydroxylation of the catecholic derivative. (A) Metabolism of DOPAC by G. pamelaeae 3C. This strain was grown in BHI medium with and without 1 mM DOPAC for 48 hr at 37°C. Metabolism was assessed using LC-MS/MS. Bars represent the mean ±the SEM concentration of the metabolite m-hydroxyphenylacetic acid (m-HPAA) resulting from direct DOPAC dehydroxylation (three biological replicates, e.g. bacterial cultures). The experiment was performed twice. (B) (+)-catechin metabolism by E. lenta A2. This strain was grown in BHI medium with and without 1 mM (+)-catechin for 48 hr at 37°C. Metabolism was assessed using high resolution LC-MS. Bars represent the mean ±the SEM mass peak area of the Extracted Ion Chromatogram (EIC) for the dehydroxylated derivative 2 shown above the bar graph in B) (three biological replicates, e.g. bacterial cultures). Due to the absence of an authentic standard, integrated peak area of the high-resolution mass is displayed. The experiment was performed twice. C) Metabolism of hydrocaffeic acid by E. lenta A2. This strain was grown in BHI medium with and without 1 mM hydrocaffeic acid for 48 hr at 37°C. Metabolism was assessed using LC-MS/MS. Bars represent the mean ±the SEM concentration of m-hydroxyphenylpropionic acid (m-HPPA) resulting from the direct dehydroxylation of hydrocaffeic acid (three biological replicates, e.g. bacterial cultures). The experiment was performed twice. All data can be found in Figure 3—source data 1. Figure 3—source data 1 Metabolism data from incubations of G. pamelaeae 3C with DOPAC and from E. lenta A2 with hydrocaffeic acid and (+)-catechin (Figure 3). https://cdn.elifesciences.org/articles/50845/elife-50845-fig3-data1-v1.xlsx Download elife-50845-fig3-data1-v1.xlsx We observed complete depletion of several host and diet-derived catechols in this initial screen (Figure 3—figure supplement 1). We chose to focus on the dehydroxylation of hydrocaffeic acid, (+)-catechin, and DOPAC for further characterization, repeating the incubations with these compounds and using LC-MS/MS to confirm the production of dehydroxylated metabolites. This analysis showed that both DOPAC and hydrocaffeic acid are directly dehydroxylated by members of this library, while (+)-catechin undergoes benzyl ether reduction followed by dehydroxylation into derivative 2, as has been observed previously (Takagaki and Nanjo, 2015) (Figure 3). While (+)-catechin metabolism has been previously linked to Eggerthella (Takagaki and Nanjo, 2015), the dehydroxylation of DOPAC and hydrocaffeic acid has only been previously observed by complex gut microbiota communities (Scheline et al., 1960; Peppercorn and Goldman, 1972). The variability in these activities across closely related gut bacterial strains suggests that distinct enzymes might dehydroxylate different catechols. Gut Actinobacteria dehydroxylate individual catechols using distinct enzymes We next sought to determine the molecular basis of the dehydroxylation reactions examined above. To test the hypothesis specific rather than promiscuous enzymes were involved, we first established that dehydroxylation is an inducible activity in Gordonibacter and Eggerthella strains (Figure 4—figure supplement 1). This allowed us to use the dehydroxylase activity of cell lysates as a proxy for transcriptional induction and a means of examining dehydroxylase activity. We grew E. lenta A2 in the presence of (+)-catechin, hydrocaffeic acid, and dopamine, and grew G. pamelaeae 3C in the presence of DOPAC. We then screened each anaerobic lysate for its activity towards all of these substrates. Consistent with our prediction, each lysate quantitively dehydroxylated only the catechol substrate with which the strain had been grown. While the E. lenta lysates did not display any promiscuity (Figure 4A), cell lysate from G. pamelaeae grown in the presence of DOPAC displayed reduced activity (<45% conversion) towards hydrocaffeic acid, which structurally resembles DOPAC (Figure 4B). Overall, these results suggest that different catechol substrates induce the expression of distinct dehydroxylase enzymes that are specific in their activity and transcriptional regulation. We expected that
更多查看译文
关键词
microbial metabolism,metalloenzyme class,gut,diet-derived
AI 理解论文
溯源树
样例
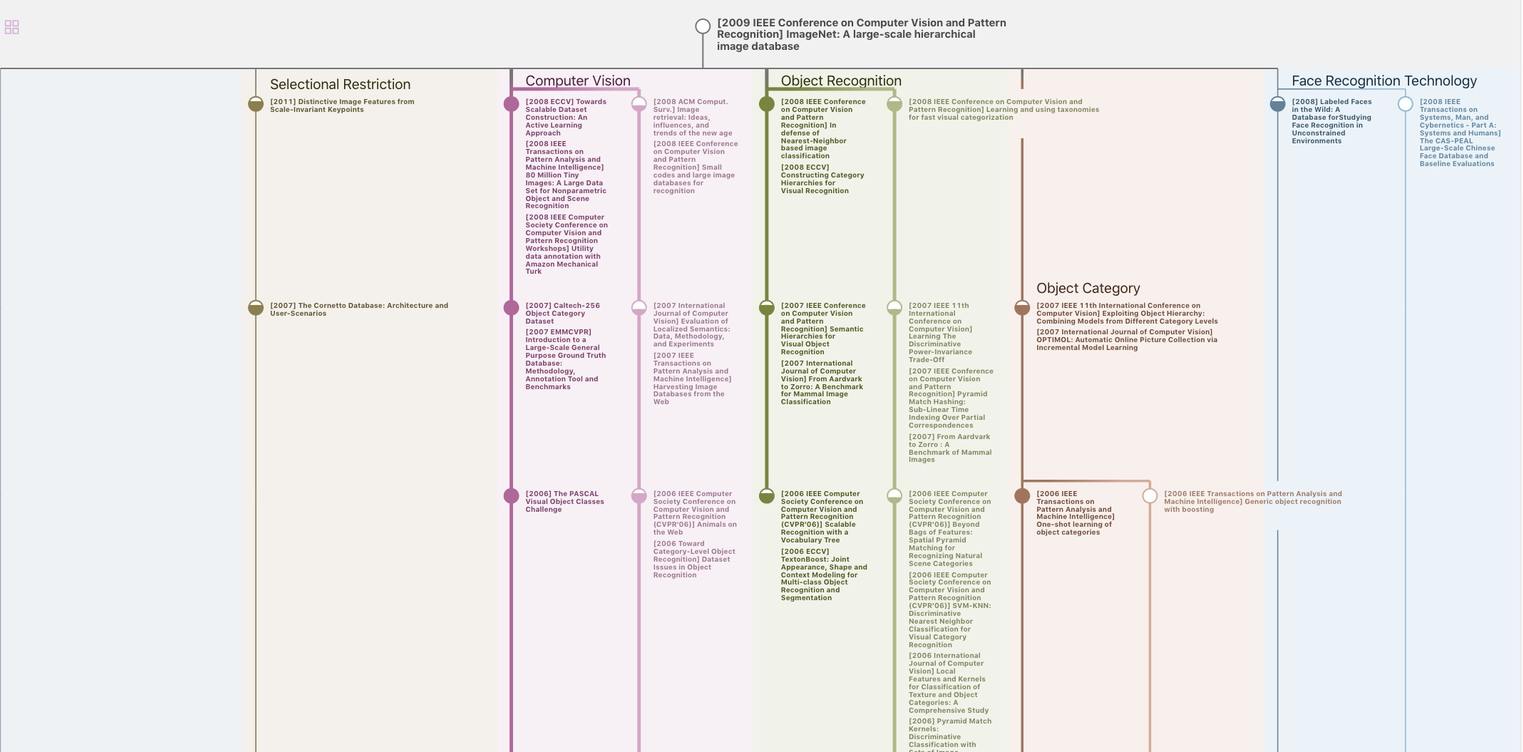
生成溯源树,研究论文发展脉络
Chat Paper
正在生成论文摘要