Hyperoxidation of mitochondrial peroxiredoxin limits H 2 O 2 ‐induced cell death in yeast
The EMBO Journal(2019)
摘要
Article7 August 2019Open Access Source DataTransparent process Hyperoxidation of mitochondrial peroxiredoxin limits H2O2-induced cell death in yeast Gaetano Calabrese Gaetano Calabrese orcid.org/0000-0002-2212-5280 Department for Chemistry, Institute for Biochemistry, University of Cologne, Cologne, Germany Search for more papers by this author Esra Peker Esra Peker Department for Chemistry, Institute for Biochemistry, University of Cologne, Cologne, Germany Search for more papers by this author Prince Saforo Amponsah Prince Saforo Amponsah Department for Biology, Cellular Biochemistry, University of Kaiserslautern, Kaiserslautern, Germany Institute of Biochemistry, University of the Saarland, Saarbruecken, Germany Search for more papers by this author Michaela Nicole Hoehne Michaela Nicole Hoehne Department for Chemistry, Institute for Biochemistry, University of Cologne, Cologne, Germany Search for more papers by this author Trine Riemer Trine Riemer Department for Chemistry, Institute for Biochemistry, University of Cologne, Cologne, Germany Search for more papers by this author Marie Mai Marie Mai Institute of Biochemistry, University of the Saarland, Saarbruecken, Germany Search for more papers by this author Gerd Patrick Bienert Gerd Patrick Bienert orcid.org/0000-0001-9345-4666 Department of Physiology and Cell Biology, Leibniz-Institute of Plant Genetics and Crop Plant Research (IPK), Gatersleben, Germany Search for more papers by this author Marcel Deponte Marcel Deponte orcid.org/0000-0003-2141-917X Department of Chemistry/Biochemistry, University of Kaiserslautern, Kaiserslautern, Germany Search for more papers by this author Bruce Morgan Corresponding Author Bruce Morgan [email protected] orcid.org/0000-0001-9393-1071 Institute of Biochemistry, University of the Saarland, Saarbruecken, Germany Search for more papers by this author Jan Riemer Corresponding Author Jan Riemer [email protected] orcid.org/0000-0002-7574-8457 Department for Chemistry, Institute for Biochemistry, University of Cologne, Cologne, Germany Search for more papers by this author Gaetano Calabrese Gaetano Calabrese orcid.org/0000-0002-2212-5280 Department for Chemistry, Institute for Biochemistry, University of Cologne, Cologne, Germany Search for more papers by this author Esra Peker Esra Peker Department for Chemistry, Institute for Biochemistry, University of Cologne, Cologne, Germany Search for more papers by this author Prince Saforo Amponsah Prince Saforo Amponsah Department for Biology, Cellular Biochemistry, University of Kaiserslautern, Kaiserslautern, Germany Institute of Biochemistry, University of the Saarland, Saarbruecken, Germany Search for more papers by this author Michaela Nicole Hoehne Michaela Nicole Hoehne Department for Chemistry, Institute for Biochemistry, University of Cologne, Cologne, Germany Search for more papers by this author Trine Riemer Trine Riemer Department for Chemistry, Institute for Biochemistry, University of Cologne, Cologne, Germany Search for more papers by this author Marie Mai Marie Mai Institute of Biochemistry, University of the Saarland, Saarbruecken, Germany Search for more papers by this author Gerd Patrick Bienert Gerd Patrick Bienert orcid.org/0000-0001-9345-4666 Department of Physiology and Cell Biology, Leibniz-Institute of Plant Genetics and Crop Plant Research (IPK), Gatersleben, Germany Search for more papers by this author Marcel Deponte Marcel Deponte orcid.org/0000-0003-2141-917X Department of Chemistry/Biochemistry, University of Kaiserslautern, Kaiserslautern, Germany Search for more papers by this author Bruce Morgan Corresponding Author Bruce Morgan [email protected] orcid.org/0000-0001-9393-1071 Institute of Biochemistry, University of the Saarland, Saarbruecken, Germany Search for more papers by this author Jan Riemer Corresponding Author Jan Riemer [email protected] orcid.org/0000-0002-7574-8457 Department for Chemistry, Institute for Biochemistry, University of Cologne, Cologne, Germany Search for more papers by this author Author Information Gaetano Calabrese1, Esra Peker1, Prince Saforo Amponsah2,3, Michaela Nicole Hoehne1, Trine Riemer1, Marie Mai3, Gerd Patrick Bienert4, Marcel Deponte5, Bruce Morgan *,3 and Jan Riemer *,1 1Department for Chemistry, Institute for Biochemistry, University of Cologne, Cologne, Germany 2Department for Biology, Cellular Biochemistry, University of Kaiserslautern, Kaiserslautern, Germany 3Institute of Biochemistry, University of the Saarland, Saarbruecken, Germany 4Department of Physiology and Cell Biology, Leibniz-Institute of Plant Genetics and Crop Plant Research (IPK), Gatersleben, Germany 5Department of Chemistry/Biochemistry, University of Kaiserslautern, Kaiserslautern, Germany *Corresponding author. Tel: +49-681-302-3339; E-mail: [email protected] *Corresponding author. Tel: +49-221-470-7306; E-mail: [email protected] The EMBO Journal (2019)38:e101552https://doi.org/10.15252/embj.2019101552 PDFDownload PDF of article text and main figures. Peer ReviewDownload a summary of the editorial decision process including editorial decision letters, reviewer comments and author responses to feedback. ToolsAdd to favoritesDownload CitationsTrack CitationsPermissions ShareFacebookTwitterLinked InMendeleyWechatReddit Figures & Info Abstract Hydrogen peroxide (H2O2) plays important roles in cellular signaling, yet nonetheless is toxic at higher concentrations. Surprisingly, the mechanism(s) of cellular H2O2 toxicity remain poorly understood. Here, we reveal an important role for mitochondrial 1-Cys peroxiredoxin from budding yeast, Prx1, in regulating H2O2-induced cell death. We show that Prx1 efficiently transfers oxidative equivalents from H2O2 to the mitochondrial glutathione pool. Deletion of PRX1 abrogates glutathione oxidation and leads to a cytosolic adaptive response involving upregulation of the catalase, Ctt1. Both of these effects contribute to improved cell viability following an acute H2O2 challenge. By replacing PRX1 with natural and engineered peroxiredoxin variants, we could predictably induce widely differing matrix glutathione responses to H2O2. Therefore, we demonstrated a key role for matrix glutathione oxidation in driving H2O2-induced cell death. Finally, we reveal that hyperoxidation of Prx1 serves as a switch-off mechanism to limit oxidation of matrix glutathione at high H2O2 concentrations. This enables yeast cells to strike a fine balance between H2O2 removal and limitation of matrix glutathione oxidation. Synopsis How H2O2 kills cells remains unclear. This study reveals that H2O2-mediated oxidation of the mitochondrial glutathione pool by peroxiredoxin Prx1 is a key determinant of peroxide-induced cell death in yeast. Cytosolic thiol peroxidases and catalases control the flow of H2O2 to mitochondria. Porins facilitate H2O2 flow from cytosol into mitochondria. The cytosolic antioxidative systems upregulates catalase Ctt1 expression when mitochondrial matrix antioxidative systems are impaired. Prx1 exclusively mediates H2O2-induced glutathione oxidation in the mitochondrial matrix. Hyperoxidation of Prx1 prevents oxidation of mitochondrial matrix glutathione and protects cells against H2O2-induced death. Introduction Reactive oxygen species (ROS) are an unavoidable consequence of life in an oxygen-rich environment. Once considered solely as harmful molecules, which cells seek to remove as efficiently as possible, it is now accepted that some ROS have important physiological functions. In this regard, one of the best understood ROS is H2O2, which acts as a second messenger in several key cellular signaling pathways (Sundaresan et al, 1995; Delaunay et al, 2002; Sobotta et al, 2015; Stocker et al, 2018). On the other hand, it remains unequivocal that high concentrations of H2O2 are toxic and can lead to cellular dysfunction and cell death. Presumably therefore, cells strive to tightly regulate H2O2 to permit sufficiently large fluctuations in H2O2 concentration for signaling purposes, while simultaneously preventing accumulation of H2O2 to toxic levels. Surprisingly, the exact mechanism(s) by which H2O2 leads to cell death remain poorly understood (Whittemore et al, 1995; Day et al, 2012; Uhl et al, 2015). The mediocre reactivity of H2O2 with most biological molecules argues against direct oxidation of cellular biomolecules being a principal driver of cell death, at least at physiologically relevant H2O2 concentrations (Winterbourn & Metodiewa, 1999; Stone, 2004; Winterbourn, 2008). Other possible triggers of H2O2-induced cell death have been proposed, including induction of apoptosis (Greetham et al, 2013), depletion of reduced cytosolic thioredoxins (Day et al, 2012), and disruption of redox signaling pathways (Sies, 2017), but well-defined molecular mechanisms remain largely elusive. Therefore, in this study we sought to address the molecular underpinnings of cellular H2O2 toxicity, using budding yeast as a model system. We hypothesized that mitochondrial matrix-localized processes may play an important role in H2O2 toxicity, for two reasons. First, the mitochondrial respiratory chain is thought to be a major source of cellular H2O2, and thus, the matrix is in close proximity to key sites of H2O2 production (Murphy, 2009; Quinlan et al, 2013). Specifically, the “leakage” of electrons from respiratory chain complexes to molecular oxygen leads to the generation of superoxide anions. Superoxide dismutases located in both the mitochondrial matrix (Sod2) and the cytosol/intermembrane space (Sod1) facilitate the subsequent rapid dismutation of superoxide to H2O2. Second, in comparison with the cytosol, the mitochondrial matrix appears to be poorly equipped with H2O2-detoxifying enzymes. The only enzymes in the yeast mitochondrial matrix that are known to be able to react efficiently with H2O2 are the 1-Cys peroxiredoxin, Prx1, and perhaps the glutathione peroxidase homolog, Gpx2 (Park et al, 2000; Ukai et al, 2011). Nonetheless, many aspects of H2O2 handling in the matrix remain poorly understood, for example, the efficiency of the matrix-localized H2O2 removal systems. Furthermore, the crosstalk between matrix H2O2 and different matrix redox couples is unclear, and often, conflicting results are present in the literature. For example, the exact reductive mechanisms for both Prx1 and Gpx2 remain a matter of debate, particularly regarding the specific roles of glutathione, glutaredoxins, thioredoxins and thioredoxin reductase (Pedrajas et al, 2000, 2010, 2016; Avery & Avery, 2001; Tanaka et al, 2005; Greetham & Grant, 2009). To gain further insight into matrix H2O2 handling in general and more specifically into possible mechanisms of H2O2-mediated toxicity, we employed genetically encoded probes that enable subcellular compartment-specific measurement of H2O2, the glutathione redox potential (EGSH), and Prx1 oxidation, together with biochemical assessment of cysteine redox states, transcriptome analyses and cell death assays. We found that the matrix glutathione pool is significantly more sensitive to H2O2-induced oxidation than the cytosolic glutathione pool. However, we found that H2O2-induced matrix glutathione oxidation is completely dependent upon the presence of Prx1. Deletion of PRX1 eliminated H2O2-induced oxidation of matrix glutathione and elicited a transcriptional response that increased levels of the cytosolic catalase, Ctt1, showing that cells can recognize, and respond to, impaired matrix redox homeostasis. The loss of glutathione oxidation in the matrix and the improved Ctt1-dependent H2O2-handling capacity of the cytosol synergistically rendered cells more resistant to an acute H2O2 treatment. We subsequently generated a range of matrix-targeted thiol peroxidases and mutant variants thereof, with differing abilities to transfer oxidative equivalents from H2O2 to glutathione. By replacing endogenous Prx1 with these peroxiredoxin variants, we revealed a striking correlation between matrix glutathione oxidation and cell death. In wild-type cells, we found that the degree of cell death is limited by hyperoxidation-based inactivation of Prx1 at high H2O2 levels, which restricts oxidation of the matrix glutathione pool. In summary, Prx1 hyperoxidation allows cells to strike a fine balance between H2O2 removal and limitation of mitochondrial glutathione oxidation, which is strongly predictive of cell death. Results Exogenous H2O2 elicits compartment-specific EGSH and H2O2 responses Little is known about the dynamic handling of H2O2 in the mitochondrial matrix of intact cells. We thus sought to characterize H2O2 flux inside the matrix and assess the impact of increased H2O2 on matrix reductive systems, particularly the glutathione pool. To this end, we made use of the genetically encoded fluorescent probes, roGFP2-Tsa2∆CR and Grx1-roGFP2, which allow the real-time monitoring of the basal H2O2 level and EGSH, respectively, in specific subcellular compartments (Gutscher et al, 2008; Morgan et al, 2016; Fig 1A and B). Both probes comprise a redox-sensitive green fluorescent protein (roGFP2; Hanson et al, 2004) genetically fused with a specific redox enzyme. For roGFP2-Tsa2∆CR, this is the Saccharomyces cerevisiae cytosolic typical 2-Cys peroxiredoxin, Tsa2, from which the resolving cysteine has been removed to increase the sensitivity of the probe to H2O2. In the case of Grx1-roGFP2, it is the human glutaredoxin, Grx1. The roGFP2-Tsa2ΔCR probe responds directly to H2O2, with the Tsa2ΔCR moiety serving to efficiently transfer oxidative equivalents from H2O2 to roGFP2. This probe is predominantly reduced by endogenous GSH/glutaredoxins, which directly reduce the roGFP2 moiety. RoGFP2-Tsa2ΔCR oxidation is therefore determined by rapid H2O2-driven oxidation and much slower GSH/glutaredoxin-driven reduction (Morgan et al, 2016; Roma et al, 2018). Conversely, Grx1-roGFP2 will predominantly only respond indirectly to H2O2, via H2O2-induced glutathione disulfide (GSSG) production and the concomitant increase in EGSH. The readout of roGFP-based probes is commonly presented as degree of probe oxidation to allow comparison between different experiments (OxD; for calculation, see Materials and Methods and Gutscher et al, 2008). OxDs of 0 and 1 therefore indicate fully reduced and fully oxidized roGFP2 moieties, respectively (Appendix Fig S1A; for more detailed discussion of the probe mechanisms, see, e.g., Roma et al, 2018). Figure 1. Matrix EGSH is more sensitive to H2O2 than its cytosolic counterpart A. A scheme depicting the H2O2 sensing mechanism of the peroxiredoxin-based H2O2 sensor roGFP2-Tsa2ΔCR. B. A scheme depicting the mechanism of the EGSH sensor, Grx1-roGFP2. C, D. The response of cytosolic and mitochondrial matrix-localized roGFP2-Tsa2ΔCR (C) and Grx1-roGFP2 (D) probes to bolus of exogenous H2O2 at the indicated concentrations. Probes were expressed in wild-type BY4742 yeast cells grown to exponential phase in SGal (−Leu) medium. E, F. The response of cytosolic and mitochondrial matrix-localized roGFP2-Tsa2ΔCR (E) and Grx1-roGFP2 (F) probes to 10 μM complex antimycin A (a complex III inhibitor). Probes were expressed in wild-type BY4742 yeast cells grown to exponential phase in SGal (−Leu) medium. G. The response of mitochondrial matrix-localized (left panel) and cytosolic (right panel) roGFP2-Tsa2ΔCR probes, expressed in BY4742 wild-type and Δtsa1Δtsa2 cells, to the addition of exogenous H2O2 at the indicated concentrations. Cells were grown to exponential phase in SGal (−Leu) medium. The lighter colored curves are controls, showing the probe response upon the addition of water. H. The response of mitochondrial matrix-localized (left panel) and cytosolic (right panel) roGFP2-Tsa2ΔCR probes, expressed in wild-type and Δtsa1Δtsa2 cells, to the addition of 10 μM antimycin A. Cells were grown to exponential phase in SGal (−Leu) medium. The lighter colored curves are controls showing the probe responses upon addition of 0.1% (v/v) ethanol. I. The response of a mitochondrial matrix-localized roGFP2-Tsa2ΔCR probe, expressed in wild-type and Δpor1 cells, to bolus at exogenous H2O2 at the indicated concentrations. Cells were grown to exponential phase in SGal (−Leu) medium. Lighter colored curves are controls showing the probe response upon the addition of water. J. The response of a mitochondrial matrix-localized roGFP2-Tsa2ΔCR probe, expressed in wild-type and Δpor1 cells, to the addition of 10 μM antimycin A. Cells were grown to exponential phase in SGal (−Leu) medium. Lighter colored curves are controls showing the probe response upon the addition of 0.1% (v/v) ethanol. K. Model. Cytosolic peroxiredoxins protect the mitochondrial matrix from internal (mitochondrial) and external H2O2. Data information: In all panels, OxD refers to the degree of sensor oxidation. Error bars represent the standard deviation (n = 3 biological replicates, with cells obtained from three independent cultures for every strain and probe combinations. For each biological replicate, three technical replicates were performed). Significance was assessed with Student's 2-tailed, unpaired, t-test. *P < 0.05; **P < 0.01; ***P < 0.001; and ****P < 0.0001. Download figure Download PowerPoint We targeted each probe to either the cytosol or the mitochondrial matrix and monitored the probe response to the addition of exogenous H2O2 using a fluorescence plate reader-based system (Appendix Fig S1B, and Fig 1C and D). We observed that the matrix roGFP2-Tsa2∆CR probe exhibited a significantly smaller response to exogenous H2O2, applied at initial concentrations of 0.1 and 1 mM, than the cytosolic roGFP2-Tsa2∆CR probe (Fig 1C). In both subcellular compartments, the OxD of the roGFP2-Tsa2∆CR probe decreased over time in the absence of H2O2, an observation explained by the depletion of oxygen in our plate reader-based assay (Morgan et al, 2016). In contrast, upon addition of exogenous H2O2, the matrix-targeted Grx1-roGFP2 probe exhibited a significantly larger response than the cytosol-localized Grx1-roGFP2 (Fig 1D). In summary, matrix EGSH is apparently more sensitive to perturbation by exogenous H2O2, even though the roGFP2-Tsa2∆CR probe reports less H2O2 in the matrix than in the cytosol. We therefore sought to identify the cause(s) of these compartment-specific differences. Cytosolic enzymes protect the matrix against both exogenous and mitochondria-derived H2O2 The smaller roGFP2-Tsa2∆CR response in the matrix compared to the cytosol could be explained by either (i) efficient cytosolic scavenging systems as well as the two mitochondrial membranes limiting the amount of H2O2 that reaches the matrix or (ii) by more efficient scavenging of H2O2 in the matrix limiting the amount of H2O2 available to react with the probe. An initial insight into these two possibilities came from monitoring roGFP2-Tsa2∆CR and Grx1-roGFP2 probe responses to antimycin A treatment. Antimycin A is an inhibitor of respiratory chain complex III that results in release of superoxide anions on the IMS side of the inner mitochondrial membrane. Superoxide anions will be rapidly dismutated, both enzymatically and spontaneously, to H2O2. Antimycin A treatment led to a larger roGFP2-Tsa2∆CR response in the matrix compared to the cytosol, i.e., the opposite of the situation following treatment with exogenous H2O2 (Fig 1E). Antimycin A treatment also induced a small deflection of EGSH in both the matrix and the cytosol. The response in the matrix appeared to be slightly larger than in the cytosol, although the overall response was very limited in both compartments (Fig 1F). Currently, we do not understand why the comparatively strong roGFP2-Tsa2∆CR probe response upon antimycin A treatment did not result in a respective EGSH deflection. A possible hint might be the antimycin A-specific response dynamics. Compared to addition of external H2O2, antimycin A induced a comparatively late oxidation of the roGFP2-Tsa2∆CR probe without recovery. In conclusion, the observation of opposing compartment-specific responses to antimycin A and exogenous H2O2 treatment indicates that the subcellular localization of H2O2 production/influx is an important determinant of subcellular compartment-specific H2O2 levels. Likely, cellular H2O2 scavenging enzymes significantly limit the (sub)cellular diffusion of H2O2 leading to the generation of steep intracellular H2O2 gradients (Winterbourn, 2008; Lim et al, 2015; Travasso et al, 2017). To further test this hypothesis, we monitored the response of cytosolic and matrix-localized roGFP2-Tsa2∆CR probes to exogenous peroxide in either wild-type cells or cells deleted for the genes encoding the two cytosolic typical 2-Cys peroxiredoxins, Tsa1 and Tsa2. Tsa1, in particular, is a highly abundant protein and thought to be an important cytosolic scavenger of H2O2 (Iraqui et al, 2009). In ∆tsa1∆tsa2 cells, we saw that cytosolic and matrix roGFP2-Tsa2∆CR responses (although starting from a different initial steady state) to exogenous H2O2 were much more similar than in wild-type cells (Fig 1G). These data thus further support the hypothesis that cytosolic H2O2 scavenging enzymes, including Tsa1 and Tsa2, limit the amount of exogenous H2O2 that can diffuse through the cytosol to ultimately reach the mitochondrial matrix. Interestingly, we also observed that Tsa1 and Tsa2 are important for the detoxification of mitochondria-derived H2O2, as a matrix roGFP2-Tsa2∆CR probe in ∆tsa1∆tsa2 cells responded more rapidly to antimycin A treatment than in wild-type cells (Fig 1H). Thus, release of H2O2 to the cytosol likely also constitutes a mitochondrial H2O2 detoxification pathway. We next tested whether transfer over the mitochondrial membranes contributes to a decreased roGFP2-Tsa2ΔCR response in the matrix compared to the cytosol. In other systems, it has been demonstrated that the velocity of H2O2 transfer over membranes is increased by the presence of specific transporters, e.g., aquaporin 8 in the NADPH oxidase 2-dependent signaling cascade (Bertolotti et al, 2016). The outer membrane of mitochondria (OMM) contains porins (in yeast Por1 and the less expressed Por2) that have been shown to facilitate small molecule transport (Kmita et al, 2004; Kojer et al, 2012). Indeed, we found that the response to 1 mM exogenous H2O2 of a matrix roGFP2-Tsa2ΔCR in a ∆por1 strain was decreased compared to a wild-type strain (Fig 1I). Conversely, following antimycin A treatment, the matrix roGFP2-Tsa2ΔCR probe responded more readily in ∆por1 cells than in wild-type cells, supporting the hypothesis that Por1 deletion decreases H2O2 transport to the cytosol (Fig 1J). In summary, Por1 appears to facilitate the bi-directional transport of H2O2 across the OMM. Collectively, our data indicate that cytosolic peroxiredoxins and porins in the OMM contribute a major line of defense for mitochondria from external H2O2 and support the efficient removal of mitochondria-derived H2O2 (Fig 1K). Ctt1 upregulation constitutes an “adaptive response” in matrix redox enzyme mutants We next investigated the contribution of matrix H2O2 scavenging enzymes toward regulation of matrix H2O2 level. The 1-Cys peroxiredoxin, Prx1, is likely the most important H2O2 scavenger in the yeast mitochondrial matrix. However, surprisingly, when we deleted this enzyme, the matrix roGFP2-Tsa2∆CR response to exogenous H2O2 was decreased (Fig 2A). This is counterintuitive for an enzyme that has been implicated in efficient matrix H2O2 handling. Conversely, when we assessed the matrix roGFP2-Tsa2∆CR response toward antimycin A treatment, the differences in response between wild-type and ∆prx1 cells were lost (Appendix Fig S2A). Collectively, these results suggest that defective matrix H2O2 handling might result in compensatory responses in the cytosol. To test this hypothesis, we monitored cytosolic H2O2 handling in strains lacking the matrix redox enzymes Prx1 and Trx3, which have both previously been linked to efficient H2O2 handling in the matrix (Pedrajas et al, 2000; Greetham & Grant, 2009; Gostimskaya & Grant, 2016). Interestingly, in both deletion strains cytosolic roGFP2-Tsa2ΔCR responses were attenuated compared to the wild-type cells (Fig 2B and C). Complementation of gene loss by expressing the matrix redox enzymes from a plasmid saw cytosolic roGFP2-Tsa2ΔCR responses revert to a wild-type-like situation (Fig 2D). Figure 2. Deletion of mitochondrial redox enzymes activates a cytosolic adaptive response A. The response of a mitochondrial matrix-localized roGFP2-Tsa2ΔCR probe, expressed in wild-type and Δprx1, to the addition of exogenous H2O2 at the indicated concentrations. Cells were grown in SGal (−Leu) medium and harvested at early exponential phase. Lighter colored curves are controls showing the probe response to the addition of water. B, C. The response of a cytosolic roGFP2-Tsa2ΔCR probe, expressed in wild-type, Δtrx3, and Δprx1 cells, to the addition of 0.1 mM (B) or 1 mM (C) exogenous H2O2. Cells were grown in SGal (−Leu) medium and harvested at early exponential phase. D. The response of a cytosolic roGFP2-Tsa2ΔCR probe, in Δtrx3 cells transformed with a Trx3 plasmid and Δprx1 cells transformed with a Prx1 plasmid, to the addition of 0.1 mM exogenous H2O2. Cells were grown in SGal medium lacking the appropriate amino acids for plasmid selection and harvested at early exponential phase. The light gray curve in both panels represents the wild-type control, treated with the same concentration of exogenous H2O2. E. The profile of mRNA expression of the yeast redox or redox-related enzymes in Δprx1 + empty vector cells, compared to Δprx1 + Prx1-WT cells, both grown to an early exponential phase in SGal (−Ura) medium (n = 3 biological replicates, with cells obtained from three independent cultures). Cutoff was set a log2(fold change (FC)) ± 0.32. Raw data are presented in Dataset EV1. F. The response of a cytosolic roGFP2-Tsa2ΔCR probe, in Δprx1 cells or Δctt1Δprx1 cells transformed with either an empty plasmid or a Prx1-WT plasmid to the addition of 0.1 mM exogenous H2O2. Cells were grown in SGal medium lacking the appropriate amino acids for selection and harvested in early exponential phase. G. Model. Levels of the cytosolic catalase Ctt1 increase when activity of mitochondrial redox enzymes is impaired, leading to an increased cytosolic capacity for H2O2 removal. Data information: In all panels, OxD refers to the degree of sensor oxidation. Error bars represent the standard deviation (n = 3 biological replicates, with cells obtained from three independent cultures for every strain and probe combinations. For each biological replicate, three technical replicates were performed). Significance was assessed with Student's 2-tailed, unpaired, t-test. **P < 0.01; ***P < 0.001; and ****P < 0.0001. Download figure Download PowerPoint To understand this apparent cytosolic adaptation, we performed RNA-Seq analysis on ∆prx1 cells transformed either with an empty p416TEF plasmid (∆prx1 + empty) or with a pEPT-PRX1 plasmid (∆prx1 + Prx1-WT) for the expression of wild-type Prx1 (Fig 2E, and Appendix Fig S2B and C). We found that the transcript encoding the cytosolic catalase, Ctt1, was significantly upregulated, while transcripts encoding other redox enzymes were not significantly enriched above threshold (Fig 2E and Dataset EV1). In line with a role of Ctt1 in the cytosolic “adaptive response”, the deletion of CTT1 in a Δprx1 background (Δctt1Δprx1) ablated the decreased response of cytosolic roGFP2-Tsa2ΔCR that was observed in ∆prx1 cells (Fig 2F). In line with the loss of the adaptive response, ∆prx1∆ctt1 cells exhibit a prolonged lag phase compared to either wild-type, ∆prx1, or ∆ctt1 cells during growth under chronic H2O2 stress (Appendix Fig S2D). Increased Ctt1 levels appear to be an “add-on” response as deletion of PRX1 in ∆tsa1∆tsa2 cells only conferred a small, although significantly, improved cytosolic H2O2 handling (Appendix Fig S2E). Thus, not only cytosolic redox enzymes protect the matrix from external H2O2 under “unperturbed” conditions, but also there appears to be a cytosol-based “adaptive response” if matrix redox enzyme systems are impaired. This Ctt1-dependent, NADPH-independent, response decreases the amount of exogenous H2O2 that reaches the mitochondrial matrix in cells with compromised matrix H2O2 detoxification systems (Fig 2G). Glutathione reductase activity is limiting in the matrix The experiments described above indicate that cytosolic redox enzymes significantly limit the amount of exogenous H2O2 that reaches the mitochondrial matrix. Nevertheless, matrix EGSH is still more responsive to exogenous H2O2 than cytosolic EGSH despite the lower concentration of H2O2 that reaches the matrix. We therefore wanted to gain a deeper understanding of the mechanistic basis of this difference. We reasoned that, in the matrix, either GSSG might be less efficiently reduced or H2O2 might more efficiently trigger glutathione oxidation in comparison with the situation in the cytosol. First, we assessed whether glutathione reductase (Glr1) levels are limiting in the matrix. Glr1 is dually localized to the cytosol and matrix. The two Glr1 variants are encoded by one gene and one mRNA that is translated from two different start codons, with the longer form encompassing a matrix-targeting sequence (Outten & Culotta, 2004). Deleting GLR1 resulted in a higher steady-state Grx1-roGFP2 oxidation in both compartments and a much larger response to exogenous H2O2 compared to wild-type control cells, confirming previous findings (Kojer et al, 2012; Morgan et al, 2013; Appendix Fig S3A). Notably, complementation of the Δglr1 strain with Glr1 exp
更多查看译文
关键词
cell death,yeast
AI 理解论文
溯源树
样例
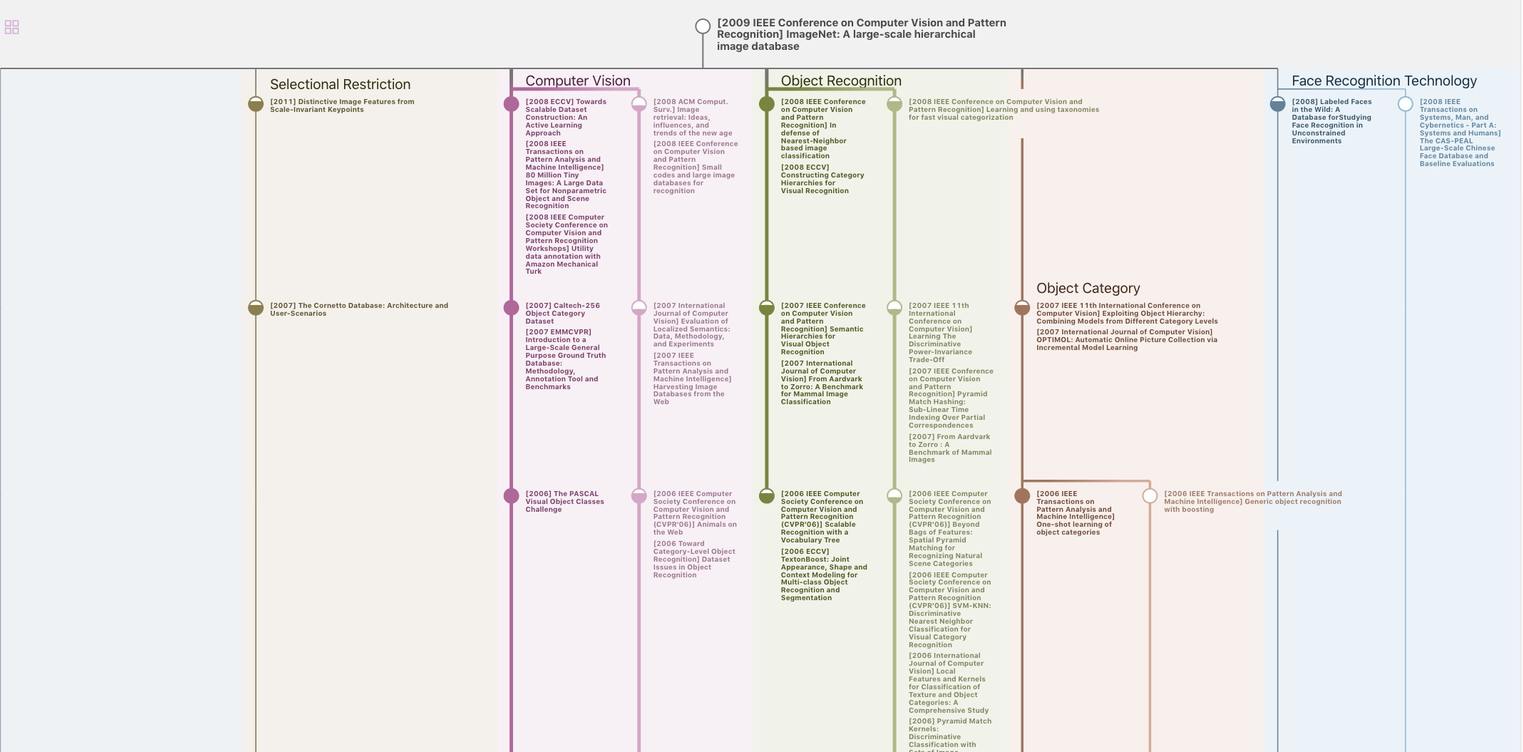
生成溯源树,研究论文发展脉络
Chat Paper
正在生成论文摘要