A common molecular mechanism underlies the role of Mps1 in chromosome biorientation and the spindle assembly checkpoint
EMBO Reports(2020)
摘要
Article19 April 2020free access Transparent process A common molecular mechanism underlies the role of Mps1 in chromosome biorientation and the spindle assembly checkpoint Giorgia Benzi CRBM, University of Montpellier, CNRS, Montpellier, France Search for more papers by this author Alain Camasses IGMM, University of Montpellier, CNRS, Montpellier, France Search for more papers by this author Yoshimura Atsunori Institute of Molecular and Cellular Biosciences, The University of Tokyo, Tokyo, Japan Search for more papers by this author Yuki Katou Institute of Molecular and Cellular Biosciences, The University of Tokyo, Tokyo, Japan Search for more papers by this author Katsuhiko Shirahige Institute of Molecular and Cellular Biosciences, The University of Tokyo, Tokyo, Japan Search for more papers by this author Simonetta Piatti Corresponding Author [email protected] orcid.org/0000-0003-1958-8919 CRBM, University of Montpellier, CNRS, Montpellier, France Search for more papers by this author Giorgia Benzi CRBM, University of Montpellier, CNRS, Montpellier, France Search for more papers by this author Alain Camasses IGMM, University of Montpellier, CNRS, Montpellier, France Search for more papers by this author Yoshimura Atsunori Institute of Molecular and Cellular Biosciences, The University of Tokyo, Tokyo, Japan Search for more papers by this author Yuki Katou Institute of Molecular and Cellular Biosciences, The University of Tokyo, Tokyo, Japan Search for more papers by this author Katsuhiko Shirahige Institute of Molecular and Cellular Biosciences, The University of Tokyo, Tokyo, Japan Search for more papers by this author Simonetta Piatti Corresponding Author [email protected] orcid.org/0000-0003-1958-8919 CRBM, University of Montpellier, CNRS, Montpellier, France Search for more papers by this author Author Information Giorgia Benzi1, Alain Camasses2, Yoshimura Atsunori3, Yuki Katou3, Katsuhiko Shirahige3 and Simonetta Piatti *,1 1CRBM, University of Montpellier, CNRS, Montpellier, France 2IGMM, University of Montpellier, CNRS, Montpellier, France 3Institute of Molecular and Cellular Biosciences, The University of Tokyo, Tokyo, Japan *Corresponding author. Tel: +33 4343 59546; E-mail: [email protected] EMBO Rep (2020)21:e50257https://doi.org/10.15252/embr.202050257 PDFDownload PDF of article text and main figures. Peer ReviewDownload a summary of the editorial decision process including editorial decision letters, reviewer comments and author responses to feedback. ToolsAdd to favoritesDownload CitationsTrack CitationsPermissions ShareFacebookTwitterLinked InMendeleyWechatReddit Figures & Info Abstract The Mps1 kinase corrects improper kinetochore–microtubule attachments, thereby ensuring chromosome biorientation. Yet, its critical phosphorylation targets in this process remain largely elusive. Mps1 also controls the spindle assembly checkpoint (SAC), which halts chromosome segregation until biorientation is attained. Its role in SAC activation is antagonised by the PP1 phosphatase and involves phosphorylation of the kinetochore scaffold Knl1/Spc105, which in turn recruits the Bub1 kinase to promote assembly of SAC effector complexes. A crucial question is whether error correction and SAC activation are part of a single or separable pathways. Here, we isolate and characterise a new yeast mutant, mps1-3, that is severely defective in chromosome biorientation and SAC signalling. Through an unbiased screen for extragenic suppressors, we found that mutations lowering PP1 levels at Spc105 or forced association of Bub1 with Spc105 reinstate both chromosome biorientation and SAC signalling in mps1-3 cells. Our data argue that a common mechanism based on Knl1/Spc105 phosphorylation is critical for Mps1 function in error correction and SAC signalling, thus supporting the idea that a single sensory apparatus simultaneously elicits both pathways. Synopsis Through isolation and characterization of the new mps1-3 mutant, this study proposes that Mps1-dependent phosphorylation of Spc105 contributes to both proper chromosome segregation and the spindle assembly checkpoint in budding yeast. mps1-3 temperature-sensitive mutant fails to correct faulty kinetochore-microtubule attachments and activate the spindle assembly checkpoint. Mps1-3 is hyperactive and does not localize at kinetochores. Suppressing mutations reducing PP1 activity at kinetochores restore proper chromosome segregation and checkpoint signalling in mps1-3 mutant cells. The mps1-3 mutant is defective in Spc105 phosphorylation and Bub1 kinetochore recruitment. Artificial recruitment of Bub1 to Spc105 rescues the chromosome segregation and checkpoint defects of mps1-3 cells. Introduction Accurate chromosome segregation is a fundamental aspect of mitosis and secures the genetic stability of a cell lineage. During the mitotic cell cycle, sister chromatids that have been generated by chromosome replication in S phase must attach through their kinetochores to microtubules emanating from opposite spindle poles in M phase. This occurs through an error correction mechanism where kinetochores dynamically attach and detach from microtubules until bipolar arrangement of sister chromatids is finalised (reviewed in ref. 1). Bipolar attachment, also referred to as amphitelic attachment, leads to tension across kinetochores, which arises from the balance of pulling forces exerted by microtubules and counteracting forces exerted by sister chromatid cohesion (reviewed in ref. 2). This tug of war leads also to chromosome congression to the metaphase plate. Key to the error correction process is the evolutionary conserved CPC (chromosome passenger complex) that is composed by the Aurora B kinase and three additional centromere-targeting and activating subunits (INCENP, survivin and borealin). The CPC detects lack of kinetochore tension (e.g. in case of monotelic attachment where only one sister kinetochore is bound to microtubules or syntelic attachment where both sister kinetochores are attached to the same spindle pole) and favours amphitelic attachments by destabilising incorrect kinetochore–microtubule connections through phosphorylation of specific kinetochore substrates (reviewed in ref. 3). The Mps1 kinase is also required for the error correction pathway in many organisms 4-8. In human cells Mps1 acts in concert with Aurora B in this process 7, 9, 10. Additionally, Mps1-specific phosphorylation targets, like the kinetochore Ska complex, have been recently involved in the error correction mechanism 11. Importantly, in budding yeast Mps1 seems to operate independently of Aurora B in chromosome biorientation and its critical targets are unknown 6, 8, 12. Although the budding yeast functional orthologue of the SKA complex, the Dam1 complex, is phosphorylated by Mps1, mutations altering these phosphorylations have no impact on chromosome segregation 12, 13. The error correction process is intimately coupled to a conserved surveillance device, the spindle assembly checkpoint or SAC, that prevents sister chromatid separation and the onset of anaphase until all chromosomes are bioriented on spindle microtubules. SAC signalling fires at unattached kinetochores, where SAC proteins are recruited, and is propagated to the whole cell for inhibition of the E3 ubiquitin ligase anaphase-promoting complex (APC) bound to its activator Cdc20. A soluble protein complex called MCC (mitotic checkpoint complex) and composed of the SAC components Bub3, BubR1, Mad2 and Cdc20 is essential for APC/Cdc20 inhibition. This, in turn, prevents degradation of APC substrates, such as securin and cyclin B, thereby halting the cell cycle before the onset of anaphase (reviewed in ref. 14). The Mps1 kinase acts at the apex of the SAC signalling pathway, by phosphorylating the Knl1/Spc105 kinetochore scaffold on its MELT repeats, which in turn recruit the Bub3-Bub1 SAC complex 15-18. Subsequent binding of the SAC factor Mad1 to Bub1, together with Mps1-mediated Mad1 phosphorylation, is a prerequisite for recruitment of Mad2 to kinetochores and MCC assembly 19-23. Consistently, phosphorylation of Knl1/Spc105 MELT repeats by Mps1 is essential for SAC signalling 15, 17, 18. The Knl1/Spc105 kinetochore protein has emerged as a key factor not only as a platform for SAC signalling, but also to silence the SAC once all pairs of sister chromatids are bipolarly attached. Indeed, Knl1/Spc105 harbours at its N-terminus an “RVSF” amino acid motif that binds to PP1, which extinguishes SAC signalling at least partly by dephosphorylating Knl1/Spc105 MELT repeats 24-29. Additionally, PP1 dephosphorylates the catalytic loop of Mps1, thus further silencing SAC 30. Aurora B has also been implicated in SAC signalling. While an early model proposed that its role in the SAC would be indirect and linked to the generation of unattached kinetochores during the correction of tension-less kinetochore–microtubule attachments 31-34, increasing evidence supports a direct role of Aurora B in SAC activation from unattached kinetochores 9, 35-39. For instance, Aurora B promotes kinetochore recruitment of several SAC factors, including Mps1, and weakens PP1 binding to Knl1 through phosphorylation of Knl1 RVSF motif, suggesting that it acts upstream of SAC signalling 7, 9, 25, 31, 40. The involvement of Mps1 and Aurora B in both error correction and SAC signalling raises a crucial, yet unsolved, question: are these two pathways concomitantly elicited by a common upstream sensory device or, alternatively, they respond to distinct defects (i.e. lack of kinetochore tension and lack of kinetochore attachment)? Is it possible to activate SAC without engaging the error correction machinery and vice versa? If the molecular machineries detecting these defects were separable, it should be possible to generate hypo- or hyper-morphic mutants in the two apical kinases that selectively affect either pathway without perturbing the other. Here, we report the characterisation of a novel temperature-sensitive mps1 mutant of budding yeast (named mps1-3) that is defective in chromosome biorientation and SAC signalling. An unbiased genetic screen for extragenic suppressors of the lethality of mps1-3 cells at high temperature revealed that mutations affecting the Spc105-PP1 interface restore proper chromosome biorientation as well as a proficient SAC response. Furthermore, artificial anchoring of Bub1 near the MELT repeats of Spc105 in mps1-3 cells results in a similar rescue. Altogether, our data indicate that Mps1 promotes both error correction and SAC signalling through a common molecular mechanism based on Spc105 phosphorylation at the MELT repeats and recruitment of the Bub1 kinase to kinetochores, thereby strengthening the idea that a single sensory apparatus engages both processes. Results The novel mps1-3 mutant is defective in chromosome segregation and proficient in SPB duplication The targets of budding yeast Mps1 in the error correction pathway are unknown so far. Dissecting the function of Mps1 in kinetochore error correction is hampered by its involvement in the duplication of spindle pole bodies (SPBs) and the assembly of a bipolar spindle 41, the first step towards chromosome biorientation. We isolated the mps1-3 mutant through a random mutagenesis screen for temperature-sensitive mps1 mutants (see Materials and Methods). Haploid mps1-3 cells grew slowly at 25–30°C and were unable to form colonies at temperatures above 32°C (Fig 1A). Sequencing of the mps1-3 allele revealed a single mutation replacing the conserved serine 635 in the kinase domain by phenylalanine (Fig 1B). To characterise the primary defects underlying the temperature sensitivity of mps1-3 cells, we synchronised in G1 mps1-3 cells, together with a wild-type control and the well-characterised mps1-1 mutant 41, and released cells at the restrictive temperature of 34°C. FACS analysis of DNA contents revealed the expected bimodal distribution of DNA contents in wild-type cells, while mps1-3 and mps1-1 cells showed abroad distribution of DNA contents after cytokinesis (90–105 min), indicative of massive chromosome missegregation (Fig 1C). However, while mps1-1 cells were unable to assemble bipolar spindles due to their failure to duplicate SPBs and to activate the SAC 41, mps1-3 cells proficiently formed bipolar spindles and elongated them during anaphase (Fig 1D and Appendix Fig S1A), suggesting that mps1-3 might be a separation of function mutant specifically defective in chromosome biorientation but proficient at SPB duplication. Figure 1. The mps1-3 mutant is defective in chromosome biorientation A. Serial dilutions of stationary phase cultures of the indicated strains were spotted on YEPD and incubated at the indicated temperatures. B. Mps1 sequence alignment around Saccharomyces cerevisiae Ser635. Sc: Saccharomyces cerevisiae; Sp: Schizosaccharomyces pombe; Mm: Mus musculus: Hs: Homo sapiens; Dm: Drosophila melanogaster. C, D. Wild-type, mps1-3 and mps1-1 mutant cells were synchronised in G1 with α-factor at permissive temperature (25°C) and then released at restrictive temperature (34°C, t = 0). Cells were collected at the indicated time points for FACS analysis of DNA contents (C) and for immunofluorescence using anti-tubulin antibodies in order to score metaphase and anaphase spindles (D). Budding and nuclear division were scored on the FACS samples. E. Frequencies of chromosome loss were quantified at permissive temperature (25°C). F. Wild-type and mps1 mutant cells bearing the TetO/TetR-GFP system to mark the centromere of chromosome V 43 and expressing the SPBs marker Spc97-mCherry were synchronised as in (C, D) and arrested in anaphase through the temperature-sensitive cdc15-2 allele. At 120 and 150 min, cells were fixed for scoring chromosome V segregation (n ≥ 172). Arrowheads indicate the sister chromatids of chromosome V. Error bars: SD. N = 3. Representative images of cells for each genotype are shown on the right. Scale bar: 5 μm. G. Wild-type and mps1-3 mutant cells carrying the TetO/TetR-GFP markers for CEN5 labelling and expressing mCherry-Tub1 were grown at 25°C and then shifted to 34°C for 1 h before filming. Cells were filmed at 34°C every 2 min by time-lapse fluorescence microscopy. DIC: differential interference contrast. Scale bar: 5 μm. Download figure Download PowerPoint To quantify the mps1-3 chromosome segregation defects, we used a genetic assay based on the ability of diploid cells to mate with a haploid tester strain upon events leading to loss of one of the two copies of the MAT locus, including loss of one entire chromosome III 42. We used wild-type and homozygous mps1-1/mps1-1, mps1-3/mps1-3 diploid cells that were grown for a defined number of generations at 25°C. Already under these permissive conditions, the appearance of mating-competent cells was 1,000- to 2,000-fold higher in the mps1 mutants relative to the wild type (Fig 1E), suggesting pronounced chromosome III loss even in conditions that allow cell proliferation. To follow directly chromosome biorientation in mps1-3 haploid cells, we visualised by fluorescence microscopy the segregation of chromosome V tagged with the TetO/TetR system 1.4 kb from the centromere (CEN5-GFP) 43. Cells were also expressing the SPB marker Spc97-mCherry and carried a cdc15-2 temperature-sensitive allele to arrest cells in telophase. G1-synchronised cells were released at the restrictive temperature of 34°C, and samples were collected at 120 and 150 min from the release. As expected, near the totality of wild-type cells bioriented and properly segregated the two sister chromatids of chromosome V at opposite SPBs, while almost all mps1-1 cells underwent CEN5 mono-orientation on the single unduplicated SPB (Fig 1F). Strikingly, the vast majority of mps1-3 mutant cells displayed duplicated SPBs that separated far apart, but at 120′ the two chromatids had segregated correctly to opposite spindle poles in 42% of the cells, whereas in 41% of the cells the two chromatids had remained at the same spindle pole. Only 4% of the cells did not duplicate the SPBs, while 12% of the cells had duplicated SPBs that remained very close to each other in the mother cell, accompanied by lack of CEN5 segregation (Fig 1F). We conclude that the mps1-3 mutant, though capable to duplicate the SPBs and form bipolar spindles, is specifically defective in chromosome biorientation at 34°C. It is worth noting, however, that at higher temperatures (37°C) mps1-3 cells also failed SPB duplication and bipolar spindle assembly, suggesting that mps1-3 is a hypomorphic mutant. Centromeric and pericentromeric cohesin is crucial for chromosome biorientation, but while centromeres transiently split in metaphase upon bipolar attachment, pericentromeric cohesion resists to pulling spindle forces 43. We therefore asked if premature loss of pericentromeric cohesion could underlie the chromosome biorientation defect of mps1-3 cells. To answer this question, we tagged with the TetO/TetR system the pericentromere of chromosome V (TetO array at −13 kb from the centromere ref. 43) and analysed its behaviour in metaphase-arrested cells by fluorescence microscopy. After a pre-synchronisation in G1, cells carrying the temperature-sensitive cdc16-1 allele (compromising APC/C activity at high temperatures and arresting cells in metaphase 44) were released at 34°C. Under these conditions, sister pericentromeric regions of chromosome V did not disjoin in most mps1-3 cells, similar to wild-type and mps1-1 cells (Appendix Fig S1B). Thus, the chromosome biorientation defect of mps1-3 cells is not explained by a premature loss of pericentric cohesion. Biorientation defects could be due to lack of kinetochore–microtubule attachments, or to a failure in the correction of faulty kinetochore–microtubule connections that are not under tension. To discriminate between these two processes, we imaged live cells with GFP-tagged CEN5 and expressing Tub1-mCherry to visualise microtubules. Cells were pre-incubated at 34°C for 1 h before filming at the same temperature. Under these conditions, while 100% of wild-type cells correctly bioriented the two sister CEN5 and segregated them to opposite spindle poles (n = 201, Figs 1G and 3H), only 51.3% of mps1-3 mutant cells bioriented CEN5, whereas 48.7% of the cells did not (n = 179, Figs 1G and 3H). During the whole movie, we did not detect lagging chromosomes or CEN5 signals off the spindle in mps1-3 cells, suggesting that kinetochore–microtubule attachment is not affected. Furthermore, in contrast to ipl1 mutant cells that missegregate chromosomes mostly towards the old SPB 45, we found no such bias in mps1-3 cells under the above conditions (Fig 3H). Interestingly, however, when we filmed mps1-3 after a G1 arrest and release at 34°C (i.e. during the first cell cycle at restrictive temperature, when the pre-existing SPB is likely fully functional), we found a strong missegregation bias towards the bud (Appendix Fig S1C). This bias tends to be lost in the subsequent cell cycles (Appendix Fig S1C). From these data, we conclude that mps1-3 cells are defective in the correction of monopolar attachments, like ipl1 mutants and in agreement with previous conclusions 6. Additionally, they could alter the normal pattern of SPB asymmetric inheritance (see Discussion). The mps1-3 mutant does not engage the spindle assembly checkpoint To assess if mps1-3 cells can engage the SAC, pre-synchronised G1 cells were released at 34°C in presence of the microtubule-depolymerising drug nocodazole. FACS analysis of DNA contents showed that wild-type cells arrested as expected in metaphase with 2C DNA contents, indicative of proficient SAC signalling. Contrariwise, mps1-3 and mps1-1 cells kept progressing through the cell cycle accumulating 4C DNA contents, indicating that they cannot engage a SAC response (Fig 2A). This was confirmed by Western blot analysis of two APC substrates, the securin Pds1 and the cyclin B Clb2, which are normally degraded in anaphase and stabilised by the SAC. As expected, both proteins remained stable in wild-type cells treated with nocodazole, while they underwent proteolysis in mps1-1 and mps1-3 mutants (Fig 2B). Thus, the mps1-3 mutant is severely defective in SAC activation at high temperature. Figure 2. The mps1-3 mutant is defective in SAC signalling and does not localise Mps1 at kinetochores A. Wild-type and mps1 mutant cells were synchronised in G1 with α-factor at 25°C and then released at 34°C in the presence of nocodazole (t = 0). Cells were collected at the indicated time points for FACS analysis of DNA contents. B. Cells were treated as in (A), and after 2 h from the release, α-factor was re-added to prevent cells from undergoing a second cell cycle. Cell samples were collected at the indicated time points for Western blot analysis of the indicated proteins. Pgk1 was used as loading control. Cyc: cycling cells. C. Top: in vitro kinase assays with recombinant Mps1 and Mps1-3 purified from Escherichia coli and incubated at 34°C for the indicated times in the presence of radioactive ATP and a recombinant Spc105 N-terminal fragment (aa 1–320) as substrate. Bottom left: kinase activity was quantified on the autoradiographs by ImageJ and normalised to the levels of the full-length protein on the Coomassie blue-stained gel (right). Bottom right: 1 μg of the recombinant Mps1 kinases used for kinase assays was loaded on SDS–PAGE and stained with Coomassie blue for normalisation of kinase assays. D. Wild-type and mps1-3 cells were synchronised in G1 with α-factor at 25°C and then released at 34°C in the presence of nocodazole. Cells were collected after 90 min and fixed with formaldehyde for ChIP-seq analysis. ChIP sequence reads were normalised against sequence reads from corresponding input samples, and relative enrichment is plotted for chromosome III around the centromere (see the centromeric regions of all 16 yeast chromosomes in Appendix Fig S2). Y-axis shows enrichment values (linear scale, range is 0–10). Values below 1.5 are shown in grey, and values above 1.5 (i.e. sequences enriched in ChIP samples) are red coloured. E. Cells with the indicated genotypes were synchronised in G1 with α-factor at 25°C and then released in fresh medium at 34°C (t = 0). Cells were collected at the indicated time points for FACS analysis of DNA contents. Download figure Download PowerPoint The Mps1-3 mutant protein has enhanced kinase activity but has reduced levels at kinetochores The catalytic activity of Mps1 is essential for both chromosome biorientation and SAC activation 5, 6, 8, 15. In order to understand if the defects of the mps1-3 mutant were due to impaired Mps1 kinase activity, we performed in vitro kinase assays using recombinant Mps1 and Mps1-3 proteins purified from bacteria. We measured the ability of these proteins to auto-phosphorylate and to phosphorylate the N-terminal part of Spc105 (aa 1–320) at different times of incubation in the presence of P33γATP at 34°C. Surprisingly, the Mps1-3 catalytic activity turned out to be more elevated than that of Mps1 at all time points (Fig 2C). This was confirmed by similar kinase assays in the presence of the exogenous substrate MBP and Mps1/Mps1-3 proteins affinity-purified from yeast cells using galactose-inducible glutathione S-transferase (GST) fusion constructs 46 (Appendix Fig S2A). Contextually, Mps1-3 protein levels in yeast cells were also 2- to 3-fold higher than wild-type Mps1 and had aberrant electrophoretic mobility (Appendix Fig S2A). The elevated Mps1-3 levels correlated with a decreased proteolysis of the protein in mitosis (Appendix Fig S2B), consistent with the idea that Mps1 turnover inversely correlates to its kinase activity 47. Since Mps1 levels at kinetochores increase when its kinase activity is inactivated 7, 40, 48-50, it is conceivable that the elevated Mps1 kinase activity in mps1-3 cells accelerates Mps1 kinetochore turnover to a threshold that could be incompatible with SAC signalling and chromosome biorientation. To test this possibility, we tagged with three HA epitopes the MPS1 and mps1-3 genes at their genomic locus and checked the distribution of the corresponding proteins on chromosomes by ChIP-seq using cells pre-synchronised in G1 and released in nocodazole for 90 min at 34°C, time at which both MPS1 and mps1-3 cells were still in mitosis. Strikingly, while wild-type Mps1 clearly accumulated at the centromeric region of all 16 yeast chromosomes, Mps1-3 did not (Fig 2D and Appendix Fig S3), suggesting that its residence at kinetochores is severely impaired. To definitely ascertain if reduced kinetochore levels are the main reason for the Mps1-3 loss of function, we artificially recruited to kinetochores GFP-tagged Mps1 and Mps1-3 in cells that co-expressed the kinetochore protein Mtw1 tagged with the high affinity GFP-nanotrap (GFP-binding domain or GBD) 51. This strategy led to constitutive association of Mps1 to the kinetochore in 91.3% of the cells and of Mps1-3 in 88.5% of the cells (n = 196 and n = 209, respectively). Cells also carried the deletion of MAD2 to obliterate SAC, which would be otherwise constitutively active following Mps1 tethering to kinetochores 49, 52. Notably, anchoring Mps1-3 to Mtw1 could partially restore proper chromosome segregation at 34°C, as shown by the bimodal distribution of DNA contents by FACS analysis (Fig 2E), suggesting that the chromosome biorientation defects of mps1-3 cells are largely accounted for by decreased Mps1 kinetochore levels. A genetic screen for spontaneous suppressors of the mps1-3 mutation Since SAC is not essential in budding yeast 53, 54, we reasoned that chromosome missegregation could be the main cause of the lethality of mps1-3 cells at high temperatures, which makes this mutant a unique genetic tool to study the role of Mps1 in chromosome biorientation. To gain insights into this process, we performed an unbiased genetic screen for spontaneous mutations suppressing the temperature sensitivity of mps1-3 cells at 34°C. Classical genetic analyses allowed us to establish if the suppressing mutations were recessive or dominant, distinguish the intragenic from the extragenic suppressors (i.e. suppressing mutation inside or outside the mps1-3 gene, respectively) and assess if the extragenic suppressors were allelic to one another. Through this screen, we could isolate 27 suppressors. Out of these, 17 turned out to be intragenic while 10 were extragenic suppressors. We focused on the extragenic suppressors, which could provide valuable insights into the molecular mechanism underlying the role of Mps1 in chromosome biorientation. Through genetic analyses and whole genome sequencing, we could establish that the suppressing mutations hit three different genes (Fig 3A and B): SPC105, encoding for the kinetochore protein Spc105/Knl1; GLC7, encoding for the catalytic subunit of the phosphatase PP1; and GRR1, encoding for an F-box protein of the E3 ubiquitin ligase complex SCF. Three GRR1 suppressors out of four carried also a mutation in RSC30, encoding for a subunit of the RSC chromatin-remodelling complex 55. The basis for the suppression by the GRR1 mutations, alone or in combination with the mutation in RSC30, was not immediately clear and will be investigated in the future. Figure 3. Characterisation of the extragenic suppressors of mps1-3 mutant cells A. Serial dilutions of stationary phase cultures of the indicated strains were spotted on YEPD and incubated at 25°C and 34°C. B. List of extragenic suppressors found in the unbiased genetic screen. C. Serial dilutions of stationary phase cultures of the indicated strains were spotted on YEPD and incubated at the indicated temperatures. D. Cells with the indicated genotypes were synchronised in G1 with α-factor at 25°C and then released in fresh medium at 34°C in presence of nocodazole (t = 0). Cells were collected at the indicated time points for FACS analysis of DNA contents. E. Cells were treated as in (D) and collected at the indicated time points for Western blot analysis of Mad1-3HA. Cyc: cycling cells. F. Cells with the indicated genotypes were synchronised at 25°C and then released in fresh medium at 34°C (t = 0). Cells were collected at the indicated time points for FACS analysis of DNA contents. G, H. Cells with the indicated genotypes carrying the TetO/TetR-GFP markers for CEN5 labelling and expressing mCherry-Tub1 were grown at 25°C and then shifted to 34°C for 1 h before filming. Cells were filmed at 34°C every 2 or 4 min by time-lapse fluorescence microscopy. Chromosome V segregation errors are reported in the table (H). Representative cells are shown as examples in the montages (G). Representative montages for wild-type and mps1-3 cells are shown in Fig 1F. DIC: differential interference contrast. Scale bar: 5 μm. I. Wild-type, mps1-3, mps1-3 spc105-18 and mps1-3 GLC7-24 cells were synchronised in G1 with α-factor at 25°C and then released at 32°C in the presence of nocodazole (note that the presence of 3HA tags at the C-terminus of Mps1-3 slightly decreases the maximal temperature of suppression). Cells were collected after 90 min and fixed with formaldehyde for ChIP-seq analysis. ChIP sequence reads were normalised against sequence reads from corresponding input samples, and relative enrichment is plotted for chromosome III around the centromere (see the centromeric regions of all 16 yeast chromosomes in Appendix Fig S5). Y-axis shows enrichment values (linear scale, range is 0–10). Values below 1.5 are shown in grey, and values above 1.5 (i.e. sequences enriched in ChIP samples) are red coloured. Download figure Download PowerPoint Spc105 (Knl1 in metazoans) is a known target of Mps1 in the SAC 15. M
更多查看译文
关键词
spindle assembly checkpoint,chromosome biorientation,mps1,common molecular mechanism,molecular mechanism
AI 理解论文
溯源树
样例
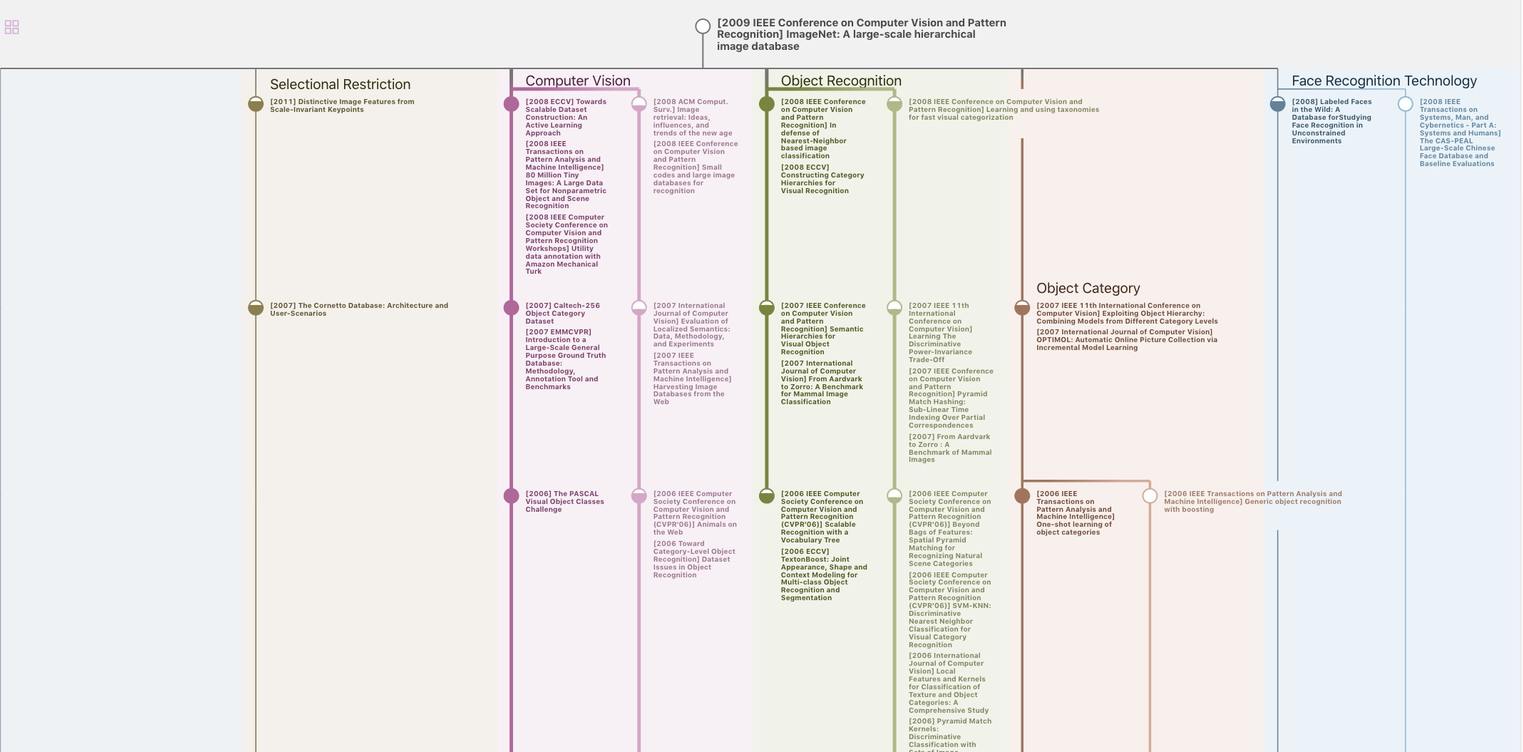
生成溯源树,研究论文发展脉络
Chat Paper
正在生成论文摘要