A Proximity-Triggered Strategy toward Transferable Proteolysis Targeting Chimeras
CCS Chemistry(2023)
摘要
Open AccessCCS ChemistryRESEARCH ARTICLES11 Jul 2022A Proximity-Triggered Strategy toward Transferable Proteolysis Targeting Chimeras Yuena Wang†, Rongtong Zhao†, Chuan Wan†, Wei Kang, Rui Wang, Chengyao Chiang, Xiaochun Guo, Qi Chang, Zhanfeng Hou, Yuxin Ye, Qinhong Luo, Ziyuan Zhou, Jianbo Liu, Shuiming Li, Dongyuan Wang, Feng Yin and Zigang Li Yuena Wang† State Key Laboratory of Chemical Oncogenomics, School of Chemical Biology and Biotechnology, Peking University Shenzhen Graduate School, Shenzhen 518055 †Y. Wang, R. Zhao, and C. Wan contributed equally to this work.Google Scholar More articles by this author , Rongtong Zhao† State Key Laboratory of Chemical Oncogenomics, School of Chemical Biology and Biotechnology, Peking University Shenzhen Graduate School, Shenzhen 518055 †Y. Wang, R. Zhao, and C. Wan contributed equally to this work.Google Scholar More articles by this author , Chuan Wan† State Key Laboratory of Chemical Oncogenomics, School of Chemical Biology and Biotechnology, Peking University Shenzhen Graduate School, Shenzhen 518055 †Y. Wang, R. Zhao, and C. Wan contributed equally to this work.Google Scholar More articles by this author , Wei Kang Shenzhen Bay Laboratory, Shenzhen 518055 Google Scholar More articles by this author , Rui Wang Pingshan Translational Medicine Center, Shenzhen Bay Laboratory, Shenzhen 518055 Google Scholar More articles by this author , Chengyao Chiang Guangdong Provincial Key Laboratory of Regional Immunity and Diseases, Shenzhen University International Cancer Center, Department of Cell Biology and Genetics, School of Medicine, Shenzhen University, Shenzhen 518055 Google Scholar More articles by this author , Xiaochun Guo State Key Laboratory of Chemical Oncogenomics, School of Chemical Biology and Biotechnology, Peking University Shenzhen Graduate School, Shenzhen 518055 Google Scholar More articles by this author , Qi Chang State Key Laboratory of Chemical Oncogenomics, School of Chemical Biology and Biotechnology, Peking University Shenzhen Graduate School, Shenzhen 518055 Google Scholar More articles by this author , Zhanfeng Hou State Key Laboratory of Chemical Oncogenomics, School of Chemical Biology and Biotechnology, Peking University Shenzhen Graduate School, Shenzhen 518055 Google Scholar More articles by this author , Yuxin Ye Pingshan Translational Medicine Center, Shenzhen Bay Laboratory, Shenzhen 518055 Google Scholar More articles by this author , Qinhong Luo State Key Laboratory of Chemical Oncogenomics, School of Chemical Biology and Biotechnology, Peking University Shenzhen Graduate School, Shenzhen 518055 Google Scholar More articles by this author , Ziyuan Zhou State Key Laboratory of Chemical Oncogenomics, School of Chemical Biology and Biotechnology, Peking University Shenzhen Graduate School, Shenzhen 518055 Google Scholar More articles by this author , Jianbo Liu Pingshan Translational Medicine Center, Shenzhen Bay Laboratory, Shenzhen 518055 Google Scholar More articles by this author , Shuiming Li Shenzhen Key Laboratory of Microbiology and Gene Engineering, Shenzhen University, Shenzhen 518055 Google Scholar More articles by this author , Dongyuan Wang *Corresponding authors: E-mail Address: [email protected] E-mail Address: [email protected] E-mail Address: [email protected] Department of Pharmacy, Union Hospital, Tongji Medical College, Huazhong University of Science and Technology, Wuhan 430030 Google Scholar More articles by this author , Feng Yin *Corresponding authors: E-mail Address: [email protected] E-mail Address: [email protected] E-mail Address: [email protected] Pingshan Translational Medicine Center, Shenzhen Bay Laboratory, Shenzhen 518055 Google Scholar More articles by this author and Zigang Li *Corresponding authors: E-mail Address: [email protected] E-mail Address: [email protected] E-mail Address: [email protected] State Key Laboratory of Chemical Oncogenomics, School of Chemical Biology and Biotechnology, Peking University Shenzhen Graduate School, Shenzhen 518055 Pingshan Translational Medicine Center, Shenzhen Bay Laboratory, Shenzhen 518055 Google Scholar More articles by this author https://doi.org/10.31635/ccschem.022.202201985 SectionsSupplemental MaterialAboutAbstractPDF ToolsAdd to favoritesDownload CitationsTrack Citations ShareFacebookTwitterLinked InEmail Over the past 20 years, great efforts have been invested in developing site-specific approaches to protein modification to dissect protein functions directly and accurately. Here, we report a proximity-triggered group transfer strategy from a sulfonium warhead to a Cysteine (Cys) residue of the target protein. With a guiding ligand, cargoes could be transferred selectively from a sulfonium center onto the Cys residue in the vicinity of their binding interface. The successful thalidomide transfer of sulfonium 1-X could be applied intracellularly for epidermal growth factor receptor degradation, highlighting the potential of group transfer strategy as a suite of chemical biology studies, including cell imaging, protein profiling, and protein degradation by simply employing different transferrable groups. Download figure Download PowerPoint Introduction Methylation of biomolecules plays an important role in the regulation of cell function. The methylation process involves an enzyme-catalyzed methyl transfer from S-Adenosyl methionine (SAM) to diverse biomolecules, including nucleic acids, proteins, lipids, and secondary metabolites. SAM has a unique methylated sulfonium center, and the methylation of other molecules happens via SAM-dependent methyltransferases (MTases).1 Notably, the sulfonium center is reasonably stable (for days) in biologically relevant conditions with glutathione and does not randomly react with biomolecules. Previous studies have shown that the replacement of the methyl on SAM with other groups like ethyl or propyl groups, allyl or propargyl groups,2 or ketone groups3 allows the transfer of these groups onto biomolecules via deliberate enzymatic engineering. More complex groups, however, are not transferable in this way. Numerous site-specific and chemo-selective protein modifications have been reported both in vitro and in vivo in the last two decades. Due to the complexity of proteins and cellular conditions, protein modifications still tend to suffer from poor selectivity, leading to the need for relatively harsh reaction conditions, slow reaction rates, and an imbalance between reactivity and selectivity.4–7 One approach for achieving site-selectivity is genetic incorporation of unnatural amino acids within proteins equipped with “bio-orthogonal” reactivity8,9 or incorporation of tag(s) for spatial recognition.10 Another approach is ligand-directed protein conjugation, triggered solely by proximity.11–15 Recently, Hamachi and colleagues16 established the use of LD chemistry to specifically label a protein of interest (POI) in living cells. Exciting applications have been derived from these protein modification methods: They provide an easy way to study post-translational modifications (PTMs) of proteins in their native environment.17 They also provide ways to attach biosensors or inhibitors onto proteins to explore complex processes inside living cells or animals.18,19 Proteolysis targeting chimeras (PROTACs)20 or specific and nongenetic inhibitor of apoptosis protein (IAP)-dependent protein erasers (SNIPERs),21 is a protein degradation strategy utilizing a heterobifunctional molecule with two recruiting ligands connected via a linker. The heterobifunctional molecule could simultaneously bind an E3 ubiquitin ligase and a POI, resulting in ubiquitination and targeted degradation of POI. PROTACs have been developed into peptide PROTACs,22,23 small molecular PROTACs,24–27 covalent PROTACs,28–33 and controllable PROTACs,34,35 and are viewed as promising approaches for drug development with successful showcases.36,37 However, optimized heterobifunctional molecules still require careful design and tedious synthesis and screening. We hypothesized that the sulfonium could achieve a proximity-triggered degrader transfer onto POIs with suitable Cysteine (Cys) in the vicinity and induces protein degradation (Figure 1). As a proof of concept study, a well-known covalent epidermal growth factor receptor (EGFR) inhibitor, Osimertinib, was chosen as the model compound. Osimertinib is a well-known drug for non-small-cell lung cancer that irreversibly inactivates the kinase activity of T790M EGFR by forming a covalent bond with a non-catalytic Cys in the adenosine 5′-triphosphate (ATP)-binding pocket.38,39 In this work, we envisioned Osimertinib-based sulfoniums and EGFR as a valuable model to explore the group transfer reaction’s potential. We first tested this model using different transferable groups, ranging from bioorthogonal groups to dyes and biotins. To test whether our sulfoniums could transfer degrader onto EGFR, we next prepare sulfoniums containing a covalent E3 ligase recruiter and Osimertinib to target EGFR. A thalidomide-type molecule was covalently and irreversibly transferred onto EGFR to achieve recruitment of the E3 ligase, CRBN. Figure 1 | Schematic presentation of biomimetic group transfer reaction for protein degradation. In this approach, the kinase inhibitor Osimertinib binds to the EGFR with high affinity, driving a group transfer reaction from a sulfonium to a Cys located on the EGFR kinase pocket. Different complex groups were transferrable and showed proof-of-concept applications in cell imaging, protein profiling, and targeted protein degradation. Download figure Download PowerPoint Experimental Methods Supplementary materials and methods for biology Sample processing of liquid chromatography with tandem mass spectrometry to determine protein modification site The protein bands of interest from 10% polyacrylamide gel were excised after electrophoresis and cut into pieces. Then the gel pieces were de-stained with a solution of 25 mM (NH4)HCO3/50% CH3CN for more than 30 min at 37 °C and reduced with freshly prepared 10 mM dithiothreitol (DTT; in 25 mM (NH4)HCO3) for 1 h at 56 °C. Next, the gel pieces were alkylated with 55 mM Iodoacetamide (in ddH2O) for 45 min at room temperature in the dark. Finally, the gel pieces were digested with 80 μL 0.0125 μg/μL trypsin (Hualishi Scientific, Beijing, China) in 50 mM (NH4)HCO3 and incubated for 12–16 h at 37 °C. Further, the digested protein fragments were extracted from gel pieces with 0.5% FA/70% CH3CN by sonicating for 3 × 5 min, combined with trypsin digestion supernatant, and dried overnight. The treated samples were used for liquid chromatography with tandem mass spectrometry (LC-MS/MS) analysis. Immunoprecipitation and ubiquitylation assay Lung carcinoma A549 cells were transfected with pcDNA3.1-HA-ubiquitin for 24 h and then treated with 20 μM 1-X (with or without 10 μM MG-132) for 6 h. Next the cells were lysed with Triton lysis buffer (0.5% Triton X-100, 1 mM ethylenediaminetetraacetic acid (EDTA), 50 mM Tris-HCl pH 7.5, 220 mM NaCl), containing protease inhibitor mixtures (1.04 mM AEBSF, 0.8 μM Aprotinin, 50 μM Bestatin, 15 μM E-64, 20 μM Leupeptin, 15 μM Pepstatin A), for 30 min on ice. Then the cell lysates were centrifuged, and the supernatants were collected. Finally, the supernatants (100 μg) were preincubated with anti-HA rabbit antibody (1 μg, ab9110, Hangzhou, China) at 4 °C overnight and spiked with 25 μL Protein A Sepharose (GE17-5280-01) for 2 h at 4 °C. The precipitates were washed with phosphate-buffered saline (PBS; 3 × 5 min) and analyzed by western blotting (WB). RNA isolation and quantitative real-time polymerase chain reaction Total RNA was extracted after incubation using Trizol reagent (Accurate Biology, Hunan, China) according to the manufacturer’s protocol. Total RNA (1 μg) was reverse transcribed to cDNA using the reverse transcriptase kit (Takara, Beijing, China) according to the manufacturer’s instructions. The mRNA levels of the target genes were detected by RT-PCR using SYBR green (Takara) in QuantStudio 5 (Applied Biosystems, Shanghai, China). Data represent three independent experiments with three technical replicates per experiment. The primers used are shown below: Human EGFR: Forward AGGCACGAGTAACAAGCTCAC, Reverse ATGAGGACATAACCAGCCACC Human GAPDH: Forward GGAGCGAGATCCCTCCAAAAT, Reverse GGCTGTTGTCATACTTCTCATGG (GAPDH = glyceraldehyde-3-phosphate dehydrogenase) Kinase enzymology assay Kinase enzymology assays were performed according to the protocols specified in the LanthaScreen® Kinase assay (Thermo Fisher Scientific, Shanghai, China). Briefly, the kinase and substrate were mixed at various inhibitor concentrations at room temperature, followed by the addition of ATP to start enzymatic reactions. After 1 h, the reactions were stopped by EDTA detection solution and then incubated with anti-Eu-antibody at room temperature for 30 min. Finally, kinase activities were measured at 665/620 nm using a plate reader (EnVision®, Perkin Elmer, Shanghai, China). Data analysis was performed with GraphPad Prism 6.0 ( https://www.graphpad.com/dl/96314/10B92408/). Results and Discussion Initial evaluation of group transfer reaction In our approach, a thiol was adducted to Osimertinib via Michael addition, and the resulting thioether was used for sulfonium preparation (Figure 2a). We initially evaluated the reaction between 10 mM benzyldimethylsulfonium and 50 mM Ac-Cys-OH in D2O by in situ NMR, which showed a clear transfer of benzyl group to Ac-Cys-OH (see Supporting Information Figures S1 and S2). Transfer of the azide group to protein was assessed by density functional theory (DFT) calculation ( 1-IV, Figure 2b, see Supporting Information Figure S3). Then recombinant EGFR was incubated with 1-IV in PBS; subsequently, we conducted azide-alkyne cycloaddition with the azide labeling dye reagent, DIBO-Cy5, to allow visualization by sodium dodecyl sulfate-polyacrylamide gel electrophoresis (SDS-PAGE) fluorescence scanning. Transfer selectivity was tested with other free Cys-containing proteins; no other fluorescent bands were observed, which clearly showed high selectivity of this ligand-directed group transfer reaction (Figure 2c). Figure 2 | Initial evaluation of group transfer reaction from sulfoniums to targeted Cys residues of proteins. (a) Synthetic route of Sulfoniums. (b) Chemical structure of sulfoniums containing simple groups/dyes to test the group transfer reaction (1-II is the sulfonium with R=methyl, and the methyl group could not transfer to the EGFR). Detailed molecule structure see Supporting Information Figure S4. (c) Selective transfer of 1-IV onto recombinant EGFR and subsequent azide-alkyne cycloaddition with DIBO-Cy5 (protein/1-IV 2.5/2.5 μM, pH 7.4, 37 °C, 1 h) and selective-transfer of 1-V onto recombinant EGFR (protein/1-V 2.5/25 μM, pH 7.4, 37 °C, 1 h). Download figure Download PowerPoint After confirming that an azide group with a benzyl linker showed selective transfer onto recombinant EGFR, we extended the transferable groups to biotin with different linkers (Figure 3a, allyl biotin VII, alkyl biotin VIII, and benzyl biotin IX) to test the efficiency of the transfer reaction. Recombinant EGFR was incubated with 2-IX, 1-VII, 1-VIII, and 1-IX and examined by WB. Noticeable differences in the WB band were obtained, which showed the highest transfer efficiency of the benzyl group (Figure 3b). The transferable band disappeared when excess amounts of Osimertinib or Gefitinib were added for competition (Figure 3c), supporting that a transfer reaction occurred after ligand recognition. In order to explore the reaction kinetics and stoichiometry, 10 equiv 1-IX was reacted with recombinant EGFR and assayed for WB. The WB results showed that the reaction reached saturation by 4 h, starting within a few minutes, even with 1 equiv 1-IX in PBS at 37 °C (Figure 3d). Figure 3 | Chemical properties of the group transfer reaction. (a) Chemical structure of sulfoniums containing biotin group with different linkers. (b) Transfer efficiency of different linkers. Pure recombinant EGFR was used as the blank control. 2-IX is the sulfonium with R=allyl, and the allyl group might transfer to the recombinant EGFR, used as the negative control (protein/sulfonium 2.5/12.5 μM, pH 7.4, 37 °C, 1 h). (c) Gefitinib and Osimertinib (100 μM) competitively blocked the labeling of 1-IX (10 μM) with recombinant EGFR (5 μM). (d) 2.5 μM recombinant EGFR was treated with 2.5/25 μM 1-IX for different lengths of time in PBS at 37 °C. (e) Selective transfer of 1-IX onto recombinant EGFR (protein/1-IX 2.5/2.5 μM, pH 7.4, 37 °C, 30 min). Download figure Download PowerPoint We further confirmed the selectivity of the transfer reaction with other purified kinases containing active Cys in the ATP-binding pockets, such as checkpoint kinase 2 (CHK2), fibroblast growth factor receptor (FGFR), mitogen-activated protein kinase 1 (MAPK1), by WB (Figure 3e, see Supporting Information “protein information”). Successful transfer of 1-IX was evaluated in vitro and in situ. To determine the specific modification sites, trypsin digested recombinant EGFR segments were analyzed using electrospray ionization-triple time-of-flight tandem mass spectrometry (ESI-Triple TOF-MS/MS). The MS/MS analysis indicated that 1-IX could label the recombinant EGFR Cys775 in a site-selective manner (see Supporting Information Figure S5, ions score 103). Furthermore, the MS/MS analysis suggested that other sulfoniums, such as 1-IV, could site-selectively label recombinant EGFR Cys775 (see Supporting Information Figure S6, ions score 54). Unfortunately, we did not observe a covalent bond between Cys797 and molecule 1-IX. We also conducted molecular dynamics studies by the simulation to investigate the interaction between EGFR and 1-IX (see Supporting Information Figure S7). Transferable PROTAC strategy for selective EGFR degradation Although several successful EGFR PROTACs have recently been reported,40–45 here we describe a covalent Osimertinib-based CRBN-recruiting EGFR degrader by transferable PROTAC strategy (Figure 4b). To this end, we synthesized sulfonium 1-X and analyzed protein levels of EGFR via WB to measure 1-X mediated degradation (Figure 4a). A549 cells were starved for 8 h before treatment with 1-X. We found that 1-X induced EGFR degradation in A549 cells in a dose- and time-dependent manner (Figure 4c,d). A control sulfonium 5 not containing EGFR-binding moiety failed to decrease EGFR expression level, suggesting that the EGFR-binding moiety was necessary for degrading EGFR (Figure 4e, see Supporting Information Figure S8a). Moreover, the failure of control molecules 6, 7, 8, and 9 to degrade EGFR confirmed that the degradation of EGFR was caused by the transferable group, thalidomide (Figure 4e, see Supporting Information Figure S8b–e). Figure 4 | Transferable PROTAC strategy induced EGFR degradation occurred through the ubiquitin-proteasome pathway. (a) Chemical structure of sulfoniums containing thalidomide degraders. (b) Proposed mechanism for transferable PROTACs. (c) EGFR levels in A549 cells after treatment with 1-X at the indicated concentrations for 24 h were determined by the intensity of Western bands and normalized to GAPDH. (d) EGFR levels in A549 cells after treatment with 20 μM 1-X for different lengths of time were determined by the intensity of Western bands and normalized to GAPDH. (e) Chemical structure of four negative controls in transferable PROTACs. (f) A549 cells were treated with the MLN-4924 (1 μM) or thalidomide (10 μM) competitor for 2 h before 1-X (20 μM) treatment for another 18 h, and the protein level of EGFR was determined by the intensity of WB bands, normalized to GAPDH. (g) A549 cells were treated with 20 μM 1-X with or without 10 μM MG-132 for 6 h and Immunoprecipitation-WB analysis of the ubiquitinated EGFR level. (h) The protein level of EGFR in A549 cells after treatment with 1-X for 24 h with or without MG-132 for 6 h was determined by the intensity of Western bands and normalized to GAPDH. (i) The fold changes of mRNA levels of EGFR were analyzed by quantitative RT-PCR. A549 cells were starved for 4 h and then pretreated with the MLN-4924 (1 μM) competitor for 2 h before 1-X treatment for another 12 h. Error bars represent the SD of at least three independent measurements. *P < 0.05, **P < 0.01 versus control; ns, not significant. Download figure Download PowerPoint To determine the degradation is mediated by CRBN, A549 cells were starved for 4 h and then pretreated with the MLN-4924 or thalidomide competitor for 2 h before 1-X treatment for another 18 h (Figure 4f). Then the A549 cells were treated with negative control PROTACs 1-XIII containing a methylated thalidomide moiety or 1-XIV containing a benzyl thalidomide moiety (Figure 4a, see Supporting Information Figure S9). The failure of these controls to degrade EGFR confirmed that the degradation of EGFR was caused by CRBN. To verify that 1-X induced ubiquitination of EGFR, we transiently transfected an expression vector of HA-tagged ubiquitin into A549 cells and then treated with 20 μM 1-X with or without 10 μM MG-132 [a potent cell-permeable proteasome and cysteine protease (calpain) inhibitor] for 6 h. Then the cell lysates were immunoprecipitated with an anti-HA (ubiquitin) antibody, and the precipitates were analyzed by WB with an anti-EGFR antibody to detect ubiquitinated EGFR protein. We found that the ubiquitinated EGFR levels were higher in the presence of 1-X and MG-132 than in the presence of MG-132 alone (Figure 4g). Finally, the addition of MG-132 (10 μM) could slow down the regulation of EGFR induced by 1-X and further supported the degradation was proteasome-dependent (Figure 4h). Collectively, the results suggested that 1-X induced EGFR degradation occurred via the ubiquitin-proteasome pathway. Critically, we also examined the performance of sulfonium 1-XI with a longer thalidomide linker and found it was comparatively less potential for EGFR degradation, possibly caused by the short distance between CRBN and EGFR (see Supporting Information Figure 10a). We also developed an analogue sulfonium 1-XII with a different thalidomide attachment site and achieved the same EGFR degradation potential (see Supporting Information Figure S10b). To investigate if the reduction of EGFR occurred at the protein level, we examined the mRNA levels of EGFR genes by RT-PCR. The relative changes in transcript quantities for each sample were determined by normalizing the mRNA levels of the house-keeping gene, GAPDH (Figure 4i). Sulfonium 1-IX/1-X inhibited the proliferation of A549 lung cancer cells Firstly, the kinase enzymology assay showed that 1-IX could inhibit EGFR phosphorylation, possibly due to the occupation of the kinase pocket with transferable biotin (Figure 5a, see Supporting Information Figure S11). We next evaluated the cell growth inhibition effects of 1-IX/ 1-X in A549 cells (Figure 5b). A potential contributor to the antiproliferation potency of 1-X might be due to the transferable PROTACs on EGFR. To further assess the antiproliferation effect of 1-IX and 1-X, we performed a cell cycle and apoptosis experiment. 1-IX/ 1-X obviously induced cell cycle arrest at G0/G1 phase in A549, followed by apoptosis in a dose-dependent manner (Figure 5c,d). Figure 5 | Sulfonium 1-IX/1-X inhibited the proliferation of lung cancer cells. (a) The IC50 curve showed the inhibition of EGFR with the inhibitor Osimertinib (IC50 = 61.04 nM), Gefitinib (IC50 = 0.28 nM), and 1-IX (IC50 = 121.30 nM). Representative data points were obtained from three independent experiments, each performed in duplicate. (b) Antiproliferative effects of 1-IX and 1-X in A549 cells, measured by MTT cell proliferation assay after 24 h of treatment with different concentrations of sulfoniums. (c) Regulation of cell cycle arrest by 1-IX/1-X was conducted by FACS analysis with PI staining. (d) Induction of apoptosis by 1-IX/1-X was examined by FACS analysis with PI and FITC-Annexin V staining. Error bars represent the SD of at least three independent measurements. *P < 0.05; **P < 0.01; ***P < 0.001 versus control. Download figure Download PowerPoint More targets, more potential With the highly efficient transfer method in our hands, we turned our attention to more degradation applications. Another well-established degradation strategy is a hydrophobic tagging strategy (HyT),46 which uses a hydrophobic tag to mimic a partially denatured protein folding state, recruiting chaperones to the POI, thereby leading to chaperone-mediated degradation by the proteasome (Figure 6b). To construct a sulfonium molecule that fits the application of HyT, an Adamantane group was installed onto the sulfonium center as 1-XV (Figure 6a). The transferable adamantane group served as a hydrophobic tag-induced EGFR degradation in a dose- and time-dependent manner (Figure 6c,d). Figure 6 | The potential of group transfer reaction. (a) Chemical structure of sulfonium 1-XV. (b) Proposed mechanism for HyT. (c) The protein level of EGFR in A549 cells treated with 20 μM 1-XV for different lengths of time was determined by the intensity of Western bands and normalized to GAPDH. (d) The protein level of EGFR in A549 cells treated with 1-XV at the indicated concentrations for 24 h was determined by the intensity of Western bands and normalized to GAPDH. Error bars represent the SD in three independent experiments. *P < 0.05; ***P < 0.001 versus control. Download figure Download PowerPoint Conclusion By employing a proximity-triggered strategy, we developed a site-selective group transfer reaction by selectively installing different functional groups onto a target Cys of a target protein such as EFGR for different purposes, including cell imaging, protein profiling, and targeted protein degradation. Most PROTACs are based on noncovalent, reversible ligands, possibly, for catalytic degradation.47 Obviously, both of these transferable PROTACs and other covalent PROTACs could not recycle or behave as catalysts, which are expected at least in stoichiometric concentrations to induce protein degradation. However, the covalent strategy might benefit from the indefinite affinity rendered by the covalent conjugation and kinetically favorable binary interaction. Finally, the transferable PROTAC could be applied readily with variable ligands, and a simple combination could be prepared rapidly for the initial discovery of the degradation efficiency. Supporting Information Supporting Information is available and includes supplementary figures, general information, synthetic procedures, and characterization data of all new compounds, along with computational details. Supplementary characterization data include copies of NMR spectra. Conflict of Interest The authors declare no competing interests. Funding Information We are grateful for the financial support from the Natural Science Foundation of China (grant nos. 21778009, 21977010, and 22007033); National Key Research and Development Program “Synthetic Biology” Key Special Project of China (grant no. 2018YFA0902504); China Postdoctoral Science Foundation (grant no. 2021M690220); the Natural Science Foundation of Guangdong Province (grant nos. 2020A1515010522, 2020A1515010766, 2019A1515110487, and 2019A1515111184); the Foundation for Basic and Applied Research of Guangdong Province (grant no. 2019A1515110489); and the Shenzhen Science and Technology Innovation Committee (grant nos. JCYJ20180507181527112, JCYJ201805081522131455, and JCYJ20170817172023838). Acknowledgments We acknowledge the financial support from the Beijing National Laboratory of Molecular Science open grant BNLMS20160112 and Shenzhen-Hong Kong Institute of Brain Science-Shenzhen Fundamental Research Institutions grant 2019SHIBS0004. This work is supported by the High-Performance Computing Platform of Peking University. We thank Prof. Hao Huang, Peking University Shenzhen Graduate School, for their gift of CHK2, FGFR1, and MAPK1
更多查看译文
关键词
transferable proteolysis,proximity-triggered
AI 理解论文
溯源树
样例
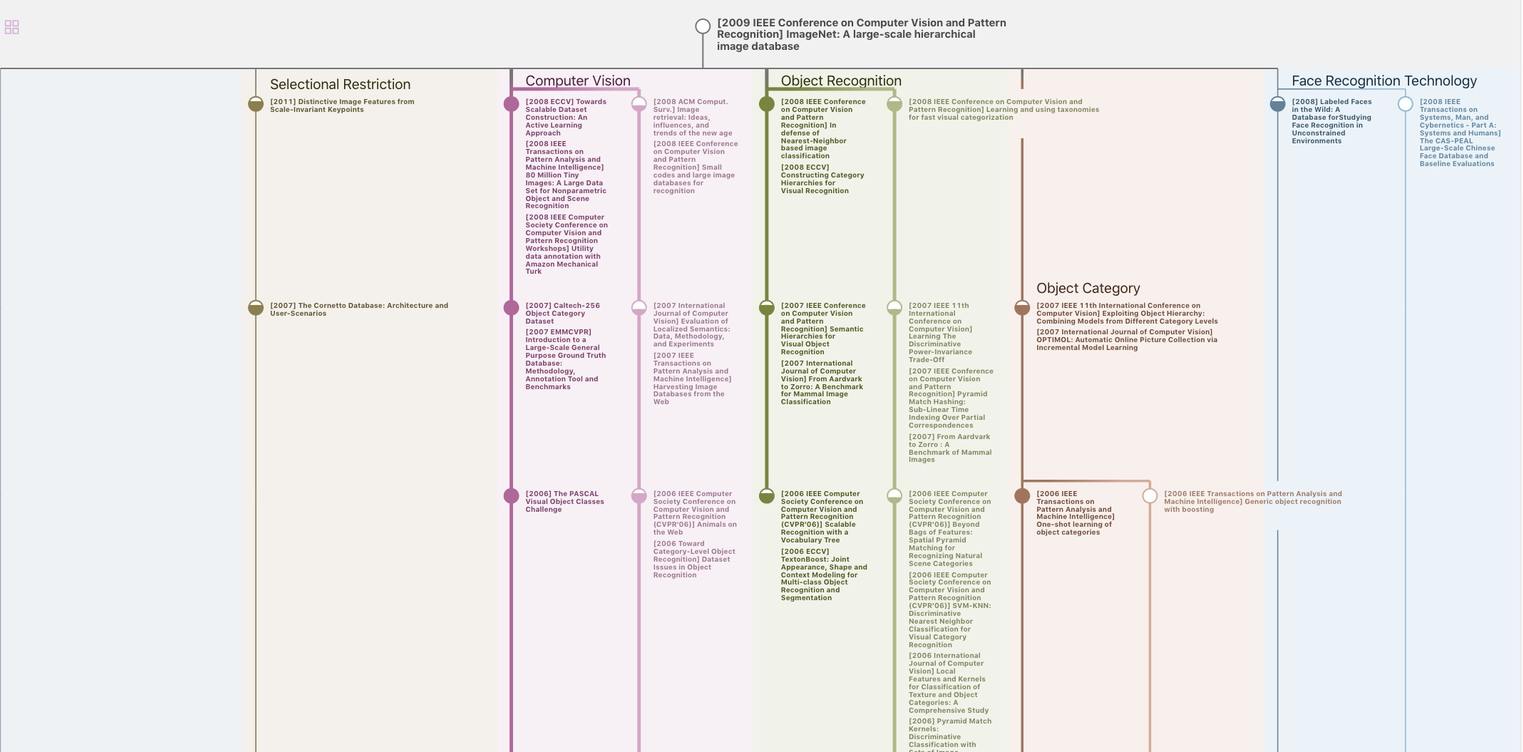
生成溯源树,研究论文发展脉络
Chat Paper
正在生成论文摘要